Gills, fins and the evolution of vertebrate paired appendages
Posted by andrewgillis, on 19 April 2016
The origin of paired fins is a major unresolved issue in vertebrate evolutionary biology, and has been a topic of debate among palaeontologists, comparative anatomists and developmental biologists for over a century. Central to any question of “evolutionary origins” is the concept of homology: the sharing of features due to common ancestry. Homology may explain the existence of shared features between organisms (historical homology – e.g. the arms of a chimpanzee and the arms of a human are homologous because these structures have been retained from a common ancestor that possessed arms), or the existence of shared features within an organism (serial homology – e.g. the vertebral elements within a human, which exhibit a range of morphologies, but which nevertheless share a common underlying ground plan) (Roth, 1984; Wagner, 1989). In either case, homology reflects a continuity of anatomical, cellular, or genetic information, and provides a useful conceptual framework for investigating the evolutionary relationship among body plan features in distantly related taxa (Van Valen, 1982).
Cartilaginous fishes (sharks, skates, rays and holocephalans) are unique among living jawed vertebrates, in that they possess a series of skeletal appendages called branchial rays that project laterally from their gill arches (Fig. 1a). These branchial rays articulate with the gill arch cartilages in a way that is broadly reminiscent of the articulation between pectoral fins or limbs and the shoulder girdle, and this similarity led the comparative anatomist Carl Gegenbaur to propose that paired fins and limbs evolved by transformation of a gill arch (Gegenbaur, 1878) (Fig. 1b, c) – a hypothesis of serial homology that remains controversial to this day. Unfortunately, the fossil record currently tells us relatively little about the stepwise acquisition of paired fins during vertebrate evolution, so we decided to address this question from a developmental perspective. We were interested in determining whether the anatomical parallels that Gegenbaur noted between the gill arches of cartilaginous fishes and fins/limbs may reflect common underlying molecular patterning mechanisms in these organ systems. To this end, we conducted a series of experiments to investigate branchial ray patterning in embryos of an oviparous (egg-laying) cartilaginous fish, the little skate, Leucoraja erinacea (see video below for an overview of skate embryonic development).
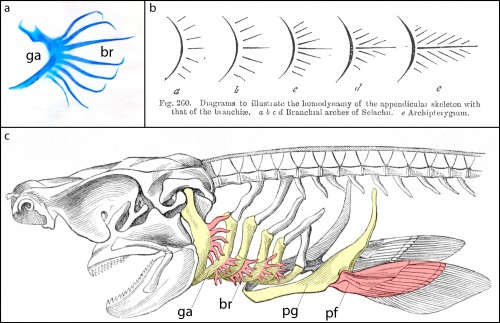
It might be helpful to first briefly introduce the gill arch, and how the gill arches of cartilaginous fishes are unique relative to those of other jawed vertebrates. All vertebrate embryos possess a bilateral series of pharyngeal arches on the sides of their developing head (Fig. 2a), and the mesenchyme within these arches gives rise to much of the craniofacial skeleton. The first pharyngeal arch is called the mandibular arch, the second is called the hyoid arch, and this is followed by a variable number of gill arches (most cartilaginous fishes have five gill arches). Primitively, the mandibular arch gave rise to the jaw skeleton, the hyoid arch gave rise to the skeletal apparatus that suspends the jaw from the braincase, and the gill arches give rise to the skeletal supports of the respiratory apparatus of the gills. These skeletal derivatives are conserved in a relatively primitive organisation in cartilaginous fishes, but are also present in other vertebrates (though often in a derived state – for example, the skeletal derivatives of the “gill arches” of mammals give rise to the laryngeal skeleton). Uniquely, though, in cartilaginous fishes, once the hyoid and gill arches have formed, they undergo a lateral expansion (Fig. 2b), and give rise to an additional set of skeletal elements – the branchial rays – that project laterally from the arches (Gillis et al., 2009). These branchial rays ultimately provide skeletal support to the fleshy flaps that protect the gills of cartilaginous fishes. As appendages, branchial rays must be patterned along the proximodistal axis (as they expand laterally) and also along the anterior-posterior axis (branchial rays exhibit a pronounced anterior-posterior polarity, and articulate proximally along the posterior margin of the hyoid and gill arch cartilages). In our paper (Gillis and Hall, 2016), we were interested in testing whether skate gill arches deploy similar axial patterning mechanisms as do fins and limbs (as would be predicted by an hypothesis of gill arch-fin/limb serial homology).
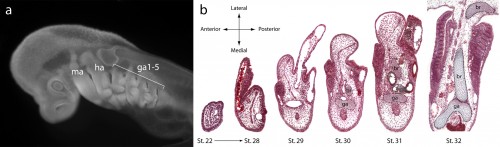
In this study, we focused our attention on the sonic hedgehog (Shh) signaling pathway, as the axial patterning function of this pathway during limb development is very well understood. Decades of experimental embryological and molecular investigation have revealed that Shh signaling is important in both anterior-posterior patterning of the limb, and in the proliferative expansion of limb skeletal progenitors. Classical chick embryo experiments by Saunders and Gasseling (1968) demonstrated that upon transplantation of donor posterior limb bud mesenchyme to the anterior region of a host limb bud, the resulting limb will form an additional set of digits that are oriented mirror-image to the normal digits (Fig. 3a). This posterior limb bud signaling centre is known as the zone of polarizing activity (ZPA). Twenty five years later, the Tabin lab demonstrated that Shh was the polarizing signal that was being secreted by the ZPA (Fig. 3b,c), and that misexpression of Shh in the anterior limb bud was sufficient to induce the ectopic, mirror-image digits noted by Saunders and Gasseling (Fib. 3d,e) (Riddle et al., 1993). In addition to this anterior-posterior patterning role, Shh signaling is also required for the expansion of limb endoskeletal progenitors, and progressively earlier loss of Shh signaling during limb development results in a progressively more profound deletions of distal limb skeletal elements (as shown, for example, by the genetic deletion of Shh from mouse limb buds, or by pharmacological manipulation of hedgehog signaling in chick and salamander) (Fig. 3f) (Towers et al., 2008; Zhu et al., 2008; Stopper and Wagner, 2007). Does Shh signaling function in a similar manner during the development of skate branchial rays?
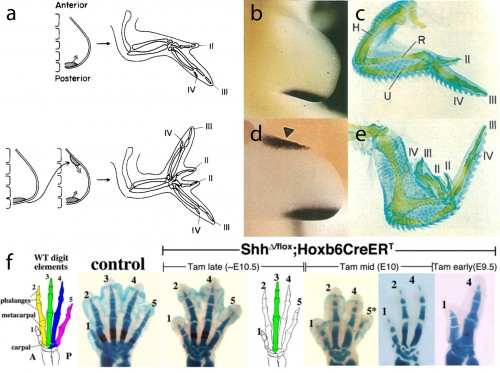
We first sought to determine whether Shh signaling components were expressed during skate gill arch development. mRNA in situ hybridization experiments revealed that, indeed, Shh is expressed in a polarized pattern during the development of the skate hyoid and gill arches – initially in the posterior epithelium of each arch, and eventually in an epithelial stripe along the leading edge of the hyoid and gill arches as they undergo lateral expansion (Fig. 4). When we looked at the expression of Ptc2 (a transcriptional readout of Shh signaling), to determine which tissues are responding to this Shh signal, we observed expression in both the distal gill arch epithelium, and in the mesenchyme beneath the Shh expression domain (Fig. 4). One key difference, of course, is that in skate gill arches, Shh is expressed in posterior-distal epithelium, while in the limb bud, Shh is expressed in posterior mesenchyme. However, in both cases, Shh signal is transduced in overlying epithelium and distal mesenchyme (so although the source of the signal is different between these appendages, the responding tissues are similar).
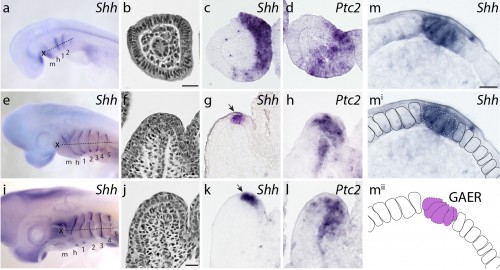
In order to determine whether Ptc2+ mesenchymal cells (i.e. mesenchymal cells responding to Shh signal) ultimately contribute to the branchial ray skeleton, we conducted a fate mapping experiment. Over the past several years, I have developed protocols for the experimental manipulation and long term in/ex ovo culture of skate embryos, and this now allows us to conduct targeted embryonic manipulations (e.g. by microinjecting and surgically manipulating specific regions of the embryo) and to focally label populations of embryonic cells, in order to trace the long-term fates of their progeny (Fig. 5a,b). For this experiments, we microinjected the lipophilic dye CM-DiI immediately subjacent to the Shh-expressing epithelium of the gill arch, so that we could assess the contribution of these cells (and their progeny) to the gill arch skeleton. CM-DiI is readily incorporated into cell membranes, and is retained in daughter cells through mitosis (although diluted somewhat with each round of cell division). Importantly, though, CM-DiI will persist through fixation and paraffin sectioning, and so upon skeletal differentiation, we can use fluorescent microscopy on thin sections to recover even very small specks of membrane-localized CM-DiI (indicating decent from mesenchymal cells that were Shh-responsive earlier in development). These experiments demonstrated that Shh-responsive gill arch mesenchyme does contribute to branchial rays (Fig. 5c), and suggest that this signaling pathway may be directly influencing the behaviour and fate of branchial ray progenitors.
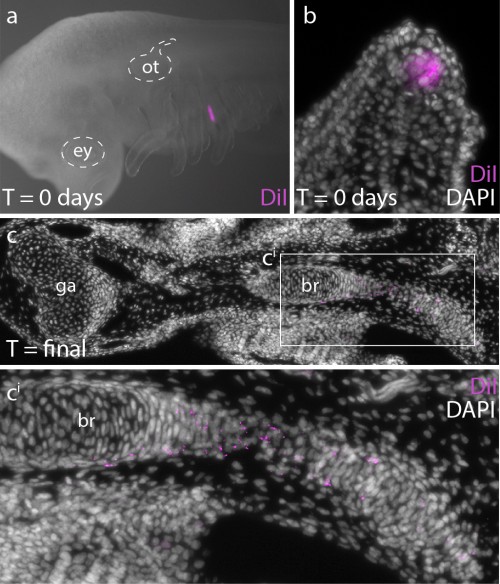
Finally, to test the function of Shh signaling during skate branchial ray development, we conducted a series of in ovo pharmacological treatments. Skate embryos develop in large, leathery egg shells, and are amenable to bath treatment by in ovo injection of small molecules. We used cyclopamine – a small molecular inhibitor of the hedgehog signaling pathway – to inhibit Shh signaling at different stages of gill arch development, and to test for stage-specific roles for Shh signaling in bronchial ray patterning. We chose three stages for treatment: stage 22 (gill arches have still formed, and Shh is expressed in posterior arch epithelium), stage 27 (gill arches are undergoing lateral expansion, with Shh signaling resolved to an epithelial stripe along the leading edge of the expanding arch) and stage 29 (just prior to the condensation of the gill arch endoskeleton). Interestingly, upon manipulation of Shh signaling at these different stages of gill arch development, we observed branchial ray defects that were broadly reminiscent of the skeletal defects observed upon manipulation of Shh signaling during limb development. For example, we observed that progressively earlier inhibition of Shh signaling resulted in a progressively greater deletion of branchial rays (i.e. cyclopamine treatments at stages 22 and 27 resulted in a significant reduction in the number of branchial rays on each arch, while treatment at stage 29 resulted in no significant difference in branchial ray number) (Fig. 6a). We also observed that cyclopamine treatment at stage 22 resulted in loss of anterior-posterior axis specification (i.e. the few branchial rays that did form articulate down the midline of the arch, rather than along the posterior margin of the arch) while cyclopamine treatment at stages 27 or 29 had no effect on the anterior-posterior axis (Fig. 6b). It therefore appears that, as in the limb bud, Shh signaling functions initially in skate gill arches to establish the anterior-posterior axis, and subsequently to maintain proliferative expansion of branchial ray endoskeletal progenitors.
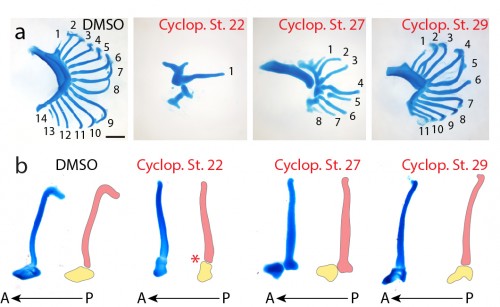
So what does this shared role for Shh signaling in gill arch and limb bud patterning mean? It is possible that limbs share a patterning mechanism with gill arches because these structures are, indeed, transformational homologues (i.e. fins and limbs evolved by transformation of a gill arch in an ancestral vertebrate, as proposed by Gegenbaur). However, it may also be that gill arches and fins/limbs have independently recruited a deeply conserved “core” appendage patterning mechanism (i.e. parallel evolution, leading to serial homology), or that gill arches and fins/limbs are convergently using the Shh signaling pathway for similar purposes. Only palaeontological data can tell us about anatomical transitions, and such data are needed in order to formally test Geganbaur’s hypothesis of gill arch-paired fin transformational homology. However, it is now clear that some of the anatomical parallels that led Gegenabur to propose his gill arch hypothesis of fin origins reflect common underlying patterning mechanisms, and further investigation of the molecular basis of branchial ray patterning in cartilaginous fishes will allow us to determine whether these common mechanisms are the result of parallel evolution or convergence. I think that this study sets out an exciting path forward to address the origin and evolution of paired appendages in vertebrates, and highlights how complementary palaeontological and developmental approaches are needed in order to truly address the big, unanswered questions in vertebrate body plan evolution.
References
Gegenbaur, C (1878) Elements of Comparative Anatomy. London, UK: Macmillan.
Gillis, J.A., Dahn, R.D. and Shubin, N.H. (2009) Chondrogenesis and homology of the visceral skeleton in the little skate, Leucoraja erinacea (Chondrichthyes: Batoidea). J. Morphol. 270, 628-643.
Gillis, J.A. and Hall, B.K. (2016) A shared role for sonic hedgehog signalling in patterning chondrichthyan gill arch appendages and tetrapod limbs. Development 143, 1313-1317.
Owen, R. (1866) Anatomy of Vertebrates I. Fishes and Reptiles. London, U.K.: Longmans, Green, and Co.
Riddle, R.D., Johnson, R.L., Laufer, E. and Tabin, C.J. (1993) Sonic hedgehog mediates the polarizing activity of the ZPA. Cell 75, 1401-1416.
Roth, V.L. (1984) On homology. Biol. J. Linn. Soc. 22, 13-29.
Saunders, J.W. and Gasseling, M.T. (1968). Ectodermal and mesenchymal interactions in the origin of limb symmetry. In Epithelial Mesenchymal Interactions (Ed. R. Fleischmajer and R. E. Billingham). Baltimore, William and Wilkins, pp. 78- 97.
Stopper, G.F. and Wagner, G.P. (2007) Inhibition of Sonic hedgehog signaling leads to posterior digit loss in Ambystoma mexicanum: parallels to natural digit reduction in urodeles. Dev. Dyn. 236, 321-331.
Towers, M., Mahood, R. Yin, Y. and Tickle, C. (2008) Integration of growth and specification in chick wing digit patterning. Nature 452, 882-886.
Van Valen, L.M. (1982) Homology and causes. J. Morphol. 173, 305-312.
Wagner, G.P. (1989) The biological homology concept. Annu. Rev. Ecol. Syst. 20, 51-69.
Zhu, J., Nakamura, E., Nguyen, M.T., Bao, X., Akiyama, H. and Mackem, S. (2008) Uncoupling sonic hedgehog control of pattern and expansion of the developing limb bud. Dev. Cell. 14, 624-632.