Is it in yet? How the direct transdifferentiation of glia-to-neurons ensures nimble male mating
Posted by Carla Lloret-Fernandez, on 25 March 2021
By Rachel Bonnington, Carla Lloret Fernández and Laura Molina García
Classically, developmental programmes were believed to be a one-way linear process in which cells progressively acquire their differentiated identities, each with a distinct and highly specialised morphology and function. Since differentiated post-mitotic identities are usually stable, differentiation was first thought to be irreversible. However, there are now many examples that appear to subvert this idea, both in nature and during forced reprogramming experiments, where differentiated cells are able to switch their identity into distinct differentiated cell types (Lambert et al. in press). This process is called transdifferentiation (Eguchi & Kodama, 1993; Selman & Kafatos,1974). In our recent paper (Molina-García et al., 2020), we describe how a glial cell transdifferentiates into a neuron in a sexually dimorphic manner to optimise male mating performance in the nematode worm C. elegans. Our findings demonstrate how genetically-programmed transdifferentiation acts as a developmental mechanism to allow flexibility in innate behaviour.
C. elegans is an excellent model for single cell studies
Two main challenges of studying transdifferentiation are that two distinct, stable identities need to be unambiguously defined, both before and after the proposed cell type conversion, and a direct lineal relationship must be established between them. This remains extremely difficult to determine in most model organisms, in which cellular lineages are highly variable and poorly defined. However, C. elegans conveniently overcomes these barriers, allowing us to study natural cellular reprogramming events in live animals. In fact, the first transdifferentiation in C. elegans was described nearly fifty years ago by Sir John Sulston, one of our scientific heroes, who observed that the rectal-epithelial cell Y converts into the PDA motor neuron (Sulston & Horvitz, 1977). This was possible because development in C. elegans is highly stereotyped and the number of cells in the adult is fixed. This allowed Sulston and colleagues to fully resolve the somatic lineage of the worm, which describes all cellular identities and their positions, for the two sexes: males and hermaphrodites (Sulston & Horvitz, 1977; Sulston et al. 1980; Sulston et al. 1983). C. elegans is also transparent, and transgenics are readily made, so cells can be imaged at a single-cell level, making it possible to unambiguously follow cell-type conversion events. No wonder then, that C.elegans is our model organism of choice in the Barrios and Poole labs at UCL!
PHso1 is a glial cell that changes identity in the worm
Previous work from the Barrios and Poole labs described how a pair of glial cells, called amphid socket (AMso) cells, undergo an identity change into neurons in males, providing the second example of transdifferentiation in the worm. The AMso cells form the cuticular pore of the amphid chemosensory organ in the head of the worm that allows sensory neurons to contact and respond to the external world. In this case, transdifferentiation occurs alongside asymmetric cell division that leads to a self-renewal of the AMso glial cells and the production of a previously unidentified male-specific pair of interneurons, the MCMs (Sammut et al. 2015). The AMso cells retain their structural role in forming the socket of the amphid, while the MCM neurons are involved in male-specific learning.
Following on from our discovery of this glia-to-neuron transdifferentiation, we were interested to examine the sensory pore of the equivalent sensory organ in the tail of worms, the phasmid sensillum. Unlike the amphid, the phasmid is made up of two pairs of socket cells (the sister cells PHso1 and PHso2), which together form a bilayer hollow pore in the cuticle. Again, during his description of the C. elegans lineage, John Sulston noted a difference between the phasmid sockets in males and hermaphrodites, observing that in adult males, PHso1 cells appear to retract from the hypodermis, and that they contain basal bodies (a structural component of cilia – in C. elegans, the only ciliated cells are sensory neurons) (Sulston, 1980). However, no other neuronal characteristics were noted and PHso1 cells continued to be classified as glia.
We were therefore hugely excited when we analysed the lin-48/OVO1 transgenic reporter, known to be expressed in PHso1, and noticed a long, axon-like projection in males, extending towards the pre-anal ganglion, a key neuronal centre in the nematode tail. This strongly suggested a neuronal identity for PHso1!
Could this be the smoking gun we were looking for, proving that in males the anterior-most phasmid socket glial cells (PHso1) were in fact being transformed into neurons? To determine this, we examined these lin-48-expressing cells during different developmental stages in males by time-lapse and compared them to hermaphrodites. We found that during the early stages of larval development, PHso1 cells appear indistinguishable between males and hermaphrodites. During the course of male sexual maturation, PHso1s in males undergo radical remodelling from a socket-glial morphology to a neuron-like morphology. By contrast, PHso1 cells remain as sockets in hermaphrodites (see Figure), thus corroborating John Sulston’s earlier observations of sexual dimorphisms in PHso1 morphology.
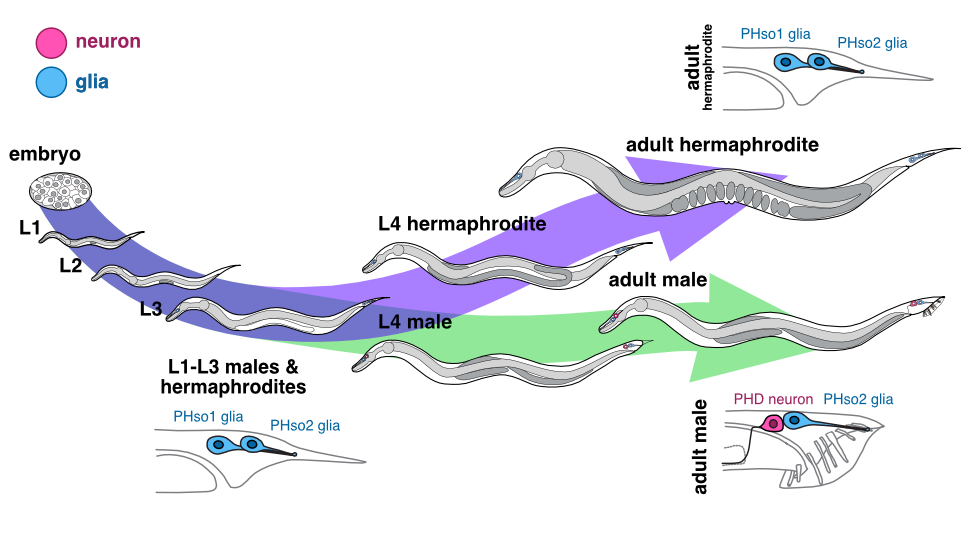
Male PHso1 glia transdifferentiate into PHD neurons
At the gene expression and ultrastructural levels, male PHso1 cells also acquire a number of uniquely neuronal characteristics, which we never observed in either the neighbouring PHso2 cells, nor the PHso1&2 cells of hermaphrodites. These include the expression of pan-neuronal genes and genes involved in neuronal transport and communication (assessed by fluorescent reporter expression) as well as the presence of synaptic vesicles, dense-core vesicles and ciliated structures (assessed by electron microscopy). Importantly, at the same stage of sexual maturation, when these neuronal markers are firing up, we see dimming of glial genes, such as the panglial microRNA mir-228 and the AMso and PHso glial subtype marker grl-2, in male PHso1s, before they fully switch off. Together, these results demonstrate that PHso1 glial cells transdifferentiate into a previously undescribed class of neurons that we call PHDs (Phasmid neuron D). This updates the anatomy of the C. elegans male, increasing the number of neurons from 385 to 387 (of which 93 are male-specific) and decreasing the total number of glia from 92 to 90.
The PHso1-to-PHD transdifferentiation seems to occur without a division. It does not require wholesale DNA replication or cell division followed by programmed cell death of one of the daughters, a common strategy used throughout development (reviewed in Conradt et al. 2016). To our surprise, however, we sometimes observed that PHso1 divides in a background-dependent manner to give rise to two apparently equivalent neurons (PHD1 and PHD2). Moreover, when we genetically manipulated the biological sex of the cell (i.e. we masculinised PHso1 in an otherwise hermaphroditic background) we observed a similar transdifferentiation of PHso1 to a PHD-like cell. This suggests that, as we previously published for the AMso, PHso1 is poised to transdifferentiate, awaiting cell-autonomous activation by the sex determination pathway.
We next sought to investigate if the molecular mechanisms known to regulate the Y-to-PDA transdifferentiation mentioned earlier could also control the transdifferentiation of the PHso1 and AMso cells. The Greenwald and Jarriault labs were the first to fully characterise the Y-to-PDA transdifferentiation (Jarriault et al., 2008). They later showed that a complex of conserved NODE-like factors (CEH-6/Oct, SEM-4/Sall and EGL-27/Mta) together with the transcription factor SOX-2, known to regulate mammalian embryonic stem cell pluripotency, are required for the initiation of the process (Kagias et al. 2012). Interestingly, these factors seem to be largely dispensable for the PHso1 and AMso cell identity switches. This could point towards independent mechanisms of transdifferentiation rather than a shared program. It will be interesting to determine if, as for Y-to-PDA, chromatin remodelling (Zuryn et al. 2014) also plays a role in AMso and PHso1 transdifferentiation, or whether transdifferentiation of these cells rely on completely different strategies. The new AMso and PHso1 cellular paradigms provide the perfect scenario for performing forward genetic screens and single-cell sequencing in order to identify, compare and contrast the molecules regulating transdifferentiation in the worm.
What is the function of PHD?
Bearing in mind that the PHD neurons arise during sexual maturation and exclusively in males, we asked ourselves what function these cells might have. First, we tried to identify the sensory stimulus that activates PHDs, by measuring neuronal activity in immobilised animals using a genetically-encoded calcium indicator. Surprisingly, we noticed spontaneous activity of the PHD neurons in the absence of any stimuli. We then realised that, despite immobilisation, some muscles near the PHD neurons were still twitching due to the defecation cycle and spasms of the spicules (the equivalent of the penis in nematodes). Perhaps the PHDs are mechanosensors and they are being activated by the internal deformations caused by those muscle contractions?
To test this idea, we measured PHD activity in worms in which muscle contractions were abolished using an inducible chemogenetic tool (an histamine-gated chloride channel transgene). Indeed, muscle silencing eliminated PHD activity, supporting our hypothesis. Furthermore, it appears that the PHDs sense muscle contractions directly and not through other neurons within the circuit because disrupting their chemical synaptic input (through mutations in genes required for synaptic transmission) did not eliminate activity. PHD neurons are thus likely to have a proprioceptive function – but which process could they be involved in?
Through reconstruction of serial electron micrographs we identified all the synaptic partners of PHD and we realised that they are not only unique to males but also highly connected to other male-specific neuronal circuits. This was highly suggestive of a role in mating. C. elegans mating behaviour is stereotyped and consists of a sequence of behavioural steps: response to a potential mate, scanning the mate’s body, turning, location of vulva, spicule insertion and ejaculation (reviewed in Barr et al., 2018). We compared the performance of each mating step in control animals and animals in which we removed PHD by laser ablation, and noticed a defect in the scanning behaviour of PHD-ablated males. In a normal mating sequence, the male scans the body of its mate moving backwards (tail-first) in a continuous manner. However, males lacking PHDs could not perform this movement smoothly, and they tended to switch directionality and pause during scanning.
As stated above, C. elegans mating behaviour is sequential and if completion of a step fails, the animal will repeat the previous step and try again. Interestingly, we noticed that wild-type males that were repeatedly unsuccessful at spicule insertion did not always return to scanning backwards in order to relocate the vulva. Instead, they performed a readjustment movement by going forwards (head-first), away from the vulva, and then returning to the vulva backwards to try to insert the spicules again (see Video 1). This was remarkable as all previously described male mating movements involve only backwards locomotion. We called this novel readjustment the ‘Molina manoeuvre’ (MM) after Laura Molina, who first observed it and to acknowledge her good eye for males!
Wildtype (Video 1) and PHD-ablated (Video 2) male worms performing Molina manoeuvres during mating with paralysed hermaphrodites (mutant for unc-51), as shown in our recent eLife paper (Molina-García et al., 2020).
When we looked specifically at MM performance, we found that animals without PHD neurons displayed discontinuous manoeuvres, often stopping at the transition from forward to backward locomotion to return to the vulva (see Video 2). Together, our data show that without intact PHD neurons, backward movement along the mating partner becomes somewhat erratic.
Are PHDs activated during backward locomotion? Consistent with the behavioural defects, we observed higher activity (i.e. rise in Ca2+ levels) in PHD during backward locomotion than during forward locomotion while scanning. The same happened during the MM, during which PHD activity also peaked just after the switch to backward locomotion. However, the highest level of PHD activation occurred during intromission. This step involves full insertion of the spicules into the mate’s vulva while sustaining backward locomotion and precedes sperm transfer. Interestingly, PHD-ablated males produced fewer cross-progeny than intact males after a single mating encounter. This suggests that intact PHDs may increase the efficiency of sperm transfer by controlling the male’s posture during intromission, which would be consistent with a putative proprioceptive role for these neurons.
Scientific significance
In summary, the previously undescribed male-specific PHD neurons are born through transdifferentiation during sexual maturation to control backward locomotion during mating. This is of high ethological relevance as the failure to complete mating implies missing a chance to reproduce and therefore failing to pass on one’s genes. Neurogenesis through transdifferentiation could facilitate strict temporal and spatial control of such finely tuned behaviours, repurposing a pre-existing cell for a newly required function, or allowing the generation of a cell only when specific structures are already in place (i.e. to ensure correct neuronal wiring).
Importantly, this is the second example of neurons arising from differentiated glial cells in C. elegans, following our previous work on the AMso. This process resembles neurogenesis in the vertebrate postnatal brain, where radial glial cells produce post-mitotic neurons (reviewed in Kriegstein and Alvarez-Buylla, 2009), raising the intriguing possibility that shared mechanisms may govern glia-to-neuron transdifferentiation in the worm and vertebrate adult neurogenesis. Identifying the mechanisms that regulate these naturally occurring switches in cell identities will improve our understanding of cellular plasticity and will help develop more efficient protocols for reprogramming cells in vitro, which is widely used for cell replacement therapies. Furthermore, a deeper understanding of how locomotion is guided by self-sensory feedback could be applied to improve the execution of behavioural sequences in artificial intelligence and robotics.
References
Barr, MM., García, LR., Portman, DS. (2018) Sexual Dimorphism and Sex Differences in Caenorhabditis elegans Neuronal Development and Behavior. Genetics 208(3): 909-935; https://doi.org/10.1534/genetics.117.300294
Conradt, B., Wu, YC., Xue, D. (2016). Programmed Cell Death During Caenorhabditis elegans Development. Genetics 203(4): 1533-1562; https://doi.org/10.1534/genetics.115.186247
Eguchi, Goro; Kodama, R., 1993. Transdifferentiation. Curr. Opin. Cell Biol. 2, 1023–1028. https://doi.org/10.1016/0955-0674(93)90087-7
Jarriault, S., Schwab, Y., Greenwald, I. (2008). A Caenorhabditis elegans model for epithelial-neuronal transdifferentiation. Proceedings of the National Academy of Sciences of the United States of America, 105(10), 3790–3795. https://doi.org/10.1073/pnas.0712159105
Kagias, K., Ahier, A., Fischer, N., Jarriault, S. (2012). Members of the NODE (Nanog and Oct4-associated deacetylase) complex and SOX-2 promote the initiation of a natural cellular reprogramming event in vivo. Proceedings of the National Academy of Sciences of the United States of America, 109(17), 6596–6601. https://doi.org/10.1073/pnas.1117031109
Kriegstein, A., Alvarez-Buylla, A. (2009). The glial nature of embryonic and adult neural stem cells. Annual review of neuroscience, 32, 149–184. https://doi.org/10.1146/annurev.neuro.051508.135600
Lambert, J., Lloret-Fernández, C., Laplane, L., Poole, R. J. & Jarriault, S. (in press). On the origins and conceptual frameworks of natural plasticity – lessons from single cell models in C. elegans. Current Trends in Developmental Biology.
Molina-García, L., Lloret-Fernández, C., Cook, SJ., Kim, B., Bonnington, RC., Sammut, M., O’Shea, JM., Gilbert, SP., Elliott, DJ., Hall, DH., Emmons, SW., Barrios, A. & Poole, RJ. (2020). Direct glia-to-neuron transdifferentiation gives rise to a pair of male-specific neurons that ensure nimble male mating. Elife 9:e48361. https://doi:10.7554/eLife.48361
Sammut, M., Cook, S. J., Nguyen, K., Felton, T., Hall, D. H., Emmons, S. W., Poole, R. J., & Barrios, A. (2015). Glia-derived neurons are required for sex-specific learning in C. elegans. Nature, 526(7573), 385–390. https://doi.org/10.1038/nature15700
Selman, K., Kafatos, F.C., 1974. Transdifferentiation in the labial gland of silk moths: is DNA required for cellular metamorphosis? Cell Differ. 3, 81–94. https://doi.org/10.1016/0045-6039(74)90030-X
Sulston, J. E. & Horvitz, H. R. (1977). Post-embryonic cell lineages of the nematode, Caenorhabditis elegans. Developmental Biology, 56(1):110-156. https://doi.org/10.1016/0012-1606(77)90158-0
Sulston, J. E., Albertson, D. G., & Thomson, J. N. (1980). The Caenorhabditis elegans male: postembryonic development of nongonadal structures. Developmental biology, 78(2), 542–576. https://doi.org/10.1016/0012-1606(80)90352-8
Sulston, J. E., Schierenberg, E., White, J. G., & Thomson, J. N. (1983). The embryonic cell lineage of the nematode Caenorhabditis elegans. Developmental Biology, 100(1), 64–119. https://doi.org/10.1016/0012-1606(83)90201-4
Zuryn, S., Ahier, A., Portoso, M., White, E. R., Morin, M. C., Margueron, R., & Jarriault, S. (2014). Transdifferentiation. Sequential histone-modifying activities determine the robustness of transdifferentiation. Science (New York, N.Y.), 345(6198), 826–829. https://doi.org/10.1126/science.1255885