The people behind the papers: Amelia Joy Thompson, Sarah K Foster & Kristian Franze
Posted by the Node Interviews, on 30 November 2016
Today’s paper comes from the latest issue of Nature Neuroscience, and shows that in addition to chemical cues, mechanical signals are vital for axon pathfinding during brain development. We caught up with PI Kristian Franze from the Department of Physiology, Development and Neuroscience at the University of Cambridge, and two of the paper’s four lead authors, Amelia Joy Thompson and Sarah K Foster, both of whom are PhD students in the Franze lab.
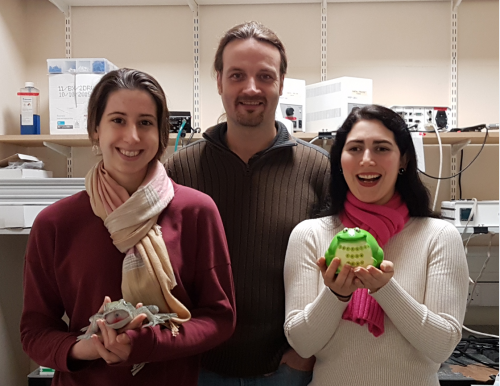
So Kristian, how did you come to establish your lab, and what are the main questions you are interested in?
KF I originally started off as a vet. However, after a short time in practice I realized that all pets have owners and that I had to spend a significant amount of time with them rather than with the animals. I quickly figured out that science is much more fun and started my PhD in neuroscience. Soon after I moved towards biophysics, and started my lab 5 years ago in the Department of Physiology, Development and Neuroscience at the University of Cambridge, after finishing my postdoc at Cambridge’s Physics Department. Our lab works at the interface of life and physical sciences; we are mainly interested in how mechanical signals control cell function in the nervous system. Particularly, we want to understand how local tissue stiffness, cellular forces, and cellular mechanosensitivity contribute to CNS development, and how changes of cells’ mechanical environment impede, for example, neuronal regeneration after spinal cord injuries.
Do you think cell mechanics are adequately appreciated in current developmental biology?
KF Definitely much more than in many other branches of biology. Forces are an old concept in developmental biology. During development, cells move all the time, and there is no motion without forces. Pretty much every developmental biologist I know appreciates cell mechanics, which makes me happy.
“During development, cells move all the time, and there is no motion without forces. Pretty much every developmental biologist I know appreciates cell mechanics”
On to the current paper: I understand it has had a long gestation?
KF That’s correct. It started some 8 years ago, when I applied for a postdoctoral fellowship with Christine Holt. That’s when I came in contact with Xenopus for the first time. Since then, we have been working on the story, had to develop a number of new experimental techniques and approaches, and to learn a lot about the mechanobiology of developing retinal ganglion cells. It went through 2 generations of highly talented postdocs and graduate students in my lab, and now I’m very happy that we finally managed to publish the paper.
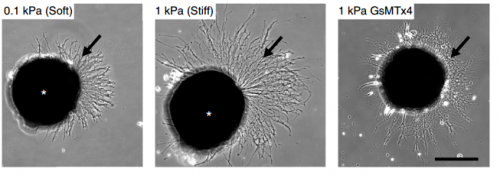
And Joy and Sarah: how did you come to be involved with this story? Were you working fairly separately, or was there a lot of collaboration?
AJT & SKF This story grew up over a long time! Joy started working on it before Sarah joined the lab, focusing on brain mechanics during development in vivo. Sarah started about a year later, working on the in vitro side. The experimental work was fairly independent, but there was a lot of scientific discussion behind the scenes among all the authors and other members of the lab. We have since started working on a collaborative project that uses Sarah’s expertise in in vitro cultures and Joy’s experience in atomic force microscopy.
Why choose the Xenopus retinal ganglion cell (RGC) as a model?
AJT & SKF The RGC model is great because it provides both a simple in vitro system and an elegant in vivo system. The structure of the optic tract is stereotypic and easily visualized, making it a brilliant system for seeing how different perturbations affect axon growth and guidance. To study in vivo brain mechanics, we needed an organism where the brain was both accessible in the embryo and developed at a rate that was practical for our measurement timeline. That’s quite a challenging set of requirements and Xenopus fit the bill nicely. Furthermore, and fortunately for us, the rate of Xenopus development can be sped up or slowed down by changing the temperature, which allows a lot of flexibility when planning experiments.
“The Xenopus retinal ganglion cell model model is great because it provides both a simple in vitro system and an elegant in vivo system”
KF Also, with Christine Holt we had a great collaborator who is a leading expert in Xenopus CNS development. This made the choice pretty easy.
AJT & SKF – On the downside, frogs are a less widely used model system and also have a complicated (allotetraploid) genome. They’re therefore less developed as a genetic model than other organisms like zebrafish, Drosophila, and even mice. Sometimes that can be quite frustrating—knockout frogs simply don’t exist, so other techniques, like morpholinos, drug treatments, or RNA/DNA injections must be used instead. Joy worked with Drosophila during her MPhil and undergraduate dissertation, so swapping to Xenopus embryos for the PhD felt a bit constraining at first! However, the Xenopus genome is known now, and CRISPR/Cas9 is showing great promise.
KF: In the end, the pros outweighed the cons by far.
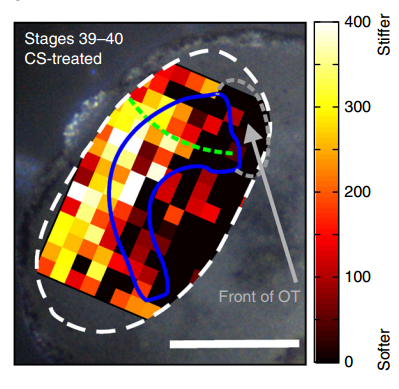
How did you go about testing the mechanics of the brain, and the responses of neurons to their mechanical environment?
AJT To measure in vivo brain mechanics, I borrowed a technique from materials science called atomic force microscopy (AFM). This is an extension of the sense of touch, just as the optical microscope is an extension of our sight. AFM uses a flexible probe (a leaf spring) to apply a set force to the brain surface; the amount by which the probe deflects (bends) is proportional to the stiffness of the tissue. It’s a bit like tapping a piece of concrete or prodding a piece of jelly. The method we used was developed in our lab over years to enable the measurement of local brain stiffness in the living embryo at cellular resolution. The AFM probe can also be used to apply a mechanical stimulus to the brain (e.g., to push on it for hours and hours) and track neuronal responses, which I did for the compression stiffening experiments.
SKF For the in vitro studies, we made compliant gels out of a polymer called polyacrylamide. Varying the ratio of two of the gel’s components allowed us precisely control the stiffness of these substrates, and we selected compositions that gave us stiffnesses near the upper and lower bounds of in vivo Xenopus brain stiffness. As brain tissue is extremely soft (comparable to cream cheese), finding the right composition was a challenge. Once we grew neuronal cultures on these substrates we were able to analyse their morphology and growth dynamics as a function of substrate stiffness using simple phase contrast microscopy.
“For the in vitro studies, we made compliant gels out of a polymer called polyacrylamide…As brain tissue is extremely soft (comparable to cream cheese), finding the right composition was a challenge.”
To further explore neuronal responses to their mechanical environment, we developed substrates containing a linear gradient in stiffness. These gradient substrates allowed us to mimic the stiffness gradients that the AFM measurements revealed in the developing brain in vivo; we could then isolate this aspect of the neuron’s environment to get a clearer idea of their mechanical response in the absence of other stimuli like chemical cues.
KF To test the importance of neuronal mechanosensitivity in vivo, we perturbed both brain stiffness and neuronal mechanotransduction in the developing embryo. While mechanically perturbing the local stiffness gradient found in the mid-diencephalon redirected axon growth, both chemically softening brain tissue and preventing neurons from detecting ‘stiff’ (either by pharmacologically blocking mechanosensitive ion channels including Piezo1 or by downregulating the expression of Piezo1) led to unusual neuronal growth patterns, and the axons never arrived at their target, the optic tectum.
Could you sum up the key results of your paper in a paragraph?
KF, AJT & SKF The key result of our paper is that neuronal growth is not only controlled by chemical signals, as it is often assumed, but also by mechanical signals. We have shown that neurons grow faster and straighter on stiffer substrates, while they slow down and grow in a more random fashion on softer ones. Piezo1, a mechanosensitive ion channel, is crucially involved in transducing the mechanical signal (here: tissue stiffness) into a response of the neuron. Developing brain tissue in vivo is mechanically highly heterogeneous and exhibits very reproducible stiffness gradients, which are likely established by changes in local cell densities in the tissue. These stiffness gradients guide neurons towards the softer side, making them turn towards their target – of course in combination with chemical guidance cues. Interfering with either tissue stiffness or neuronal mechanosensitivity was sufficient to mess up neuronal growth and pathfinding.
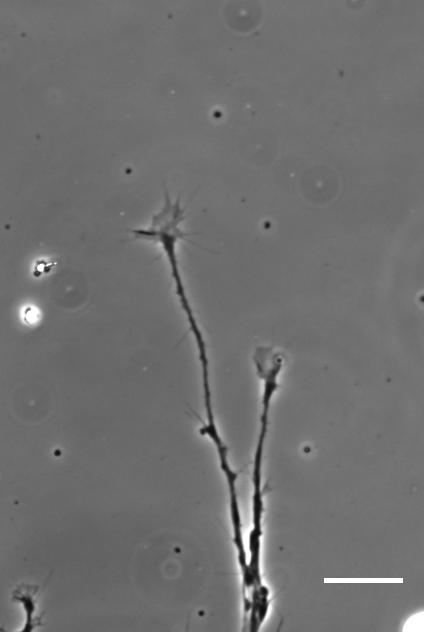
So how does a cell integrate mechanical information with the classical chemical cues? Do mechanical signals carry more weight?
SKF That’s an area we’re increasingly interested in now, and in fact is the topic of my Ph.D! Growing neurons encounter a massively complex environment. They are barraged with myriad chemical and mechanical cues and must integrate all this information to arrive at a coherent response. The growth cone – a sensory-motor apparatus at the tip of the growing neuron – is amazingly complex and how it performs this ‘analysis’ is not yet clear.
We think that chemical and mechanical signals likely work together in development. We require multiple senses in order to understand our surrounding environment completely, and likewise neurons need both chemical and mechanical information to inform their growth and development.
Additionally, once mechanical cues get past the membrane they are likely carried through similar or overlapping signal transduction cascades as chemical cues. For example both chemical and mechanical guidance signals likely affect Rho/Rac signalling to control cytoskeletal dynamics in the growth cone, which eventually determines neuronal growth. That chemical and mechanical signals converge on common pathways and signalling cascades indicates that these two types of signals likely interact in many elegant and subtle ways.
“Growing neurons encounter a massively complex environment. They are barraged with myriad chemical and mechanical cues and must integrate all this information to arrive at a coherent response”
KF I’m not sure if mechanical signals carry more weight than chemical ones. It’s probably more likely that tissue stiffness is used to modify responses to chemical cues and make the responses more robust. Topological cues (such as scratches in the surface of a culture substrate), on the other hand, which I’d also classify as mechanical signals, have long been known to be able to override chemical signals and guide growing neurons or migrating cells along them. I think this is a difficult question and we are only beginning to understand the role of mechanical signals in controlling development.
Unlike in the durotaxis of cell collectives in vitro, the neurons prefer to grow towards softer tissue. Is this just an in vivo/in vitro distinction, or do you expect this preference to vary depending on developmental context?
AJT We see this in vitro as well as in vivo, at least with Xenopus retinal ganglion cells. When bundles of retinal ganglion cell axons grow over a culture substrate with a stiffness gradient, they still tend to turn towards the softer region. Since RGC axons grow faster on stiffer substrates than they do on soft, we think this ‘mollotaxis’ is at least partly a collective effect. When a bundle of axons is exposed to a stiffness gradient, the ones on the stiffer side will grow faster than the ones on the softer side. However, as the axons are tightly coupled to each other along their length by cell adhesion molecules, the faster growing ones will be held back by the slower ones and bend towards them, i.e. towards soft. It’s analogous to plants growing in the sun: the cells in the stem pointing away from the sun grow faster than the cells on the brighter side and thus push the whole plant towards the light.
Whether this is a general property of developing neurons is still an open question. Certainly previous in vitro work has shown that different neuronal populations vary in their response to soft versus stiff substrates, so there may be an element of cell type specificity. Different neuronal populations may have different preferences. Growing axons can also change their response to the same chemical cue over time – so why not to mechanical ones too?
“Growing axons can also change their response to the same chemical cue over time – so why not to mechanical ones too?”
When doing the research, was there a particularly exciting result or eureka moment that has stayed with you?
AJT The compression stiffening experiments really stood out to me. Compression stiffening is a phenomenon where brain tissue increases in stiffness when a force is applied to it. I took advantage of this to increase the local tissue stiffness in different areas of the embryo brain in vivo and see if the RGC axons changed their growth behaviour. It turned out that they grew away from the stiffened region regardless of where the force was applied. It was amazing to see such a dramatic neuronal response to a stimulus that was purely mechanical (while all chemical guidance cues were very likely present as normal)! This was an exciting experiment, but at the same time the principle was refreshingly straightforward: what would this do if I poked it?
SKF Once we got the in vitro GsMTx-4 experiments working – where we inhibited mechanosensitive ion channels and then examined neuron morphology- the phenotype of treated axons lined up really precisely with that of axons grown on soft substrates. There was a beautifully clear correspondence between those two conditions—much better than we could have hoped for!
And what about the flipside: any particular moments or frustration and despair?
AJT On my first try of the compression stiffening experiment – which took a whole weekend, and an additional two days and nights to prepare the brains for imaging – I saw to my horror that the control specimens looked very, very wrong. It turned out to be a problem with one of our stock solutions, and thus easily fixed, but it was still extremely frustrating to repeat such a time-consuming experiment for such a simple reason. (Not to mention losing my Sundays off!)
SKF Working with the GsMTx-4 – the tarantula-venom derived peptide that blocks mechanosensitive ion channels – was quite frustrating. Batches seemed to vary in strength quite significantly and also degrade and lose strength over time, so you never quite knew what was going to happen when you put GsMTx-4 on your neurons. I killed quite a few sets of cultures by overdosing them, before I figured out how to handle it properly.
Finally, Joy and Sarah: what next for you two after this project?
SKF I’m still at the beginning of my PhD, so I’ll spend the next few years trying to gain some insight into how cells integrate chemical and mechanical cues.
AJT I am working on a follow-up to the in vivo experiments in the paper, looking in more detail at the changes in brain mechanics at different stages in development. The overarching goal is to finish writing up my PhD, as I only have a year or so left now!
“If tissue stiffness changes during pathological processes, what are the consequences for cells in the tissue?”
And Kristian, where will this work take you?
KF That’s an excellent question. This work has raised many more questions than it actually answered. We are now in the great position to choose from many fascinating questions and to work on what excites us most. We will certainly continue to work on the importance of mechanical signals for neuronal and CNS development, but will also look at regenerative processes. If tissue stiffness changes during pathological processes, what are the consequences for cells in the tissue? Also, the question you asked earlier about the integration of mechanical and chemical signals is key to understanding what is really going on during development and pathology. I guess we have enough open questions to keep us busy for quite a while.
David E Koser, Amelia J Thompson, Sarah K Foster, Asha Dwivedy, Eva K Pillai, Graham K Sheridan, Hanno Svoboda, Matheus Viana, Luciano da F Costa, Jochen Guck, Christine E Holt & Kristian Franze. 2016. Mechanosensing is critical for axon growth in the developing brain. Nature Neuroscience 19, 1592–1598.
Browse the People Behind the Papers archive here.