Live-cell analysis of plant embryogenesis: Live-cell imaging, optical manipulation, and micro-engineering technologies
Posted by Daisuke Kurihara, on 16 October 2015
In multicellular animals and plants, the single-celled zygote develops into the embryo. In the model plant Arabidopsis thaliana, the zygote divides asymmetrically to form a small cytoplasmic apical cell, which is the precursor of the proembryo, and a large vacuolated basal cell, which develops into the extra-embryonic suspensor (Figure 1). Communication between the intra-embryo (proembryo and suspensor) and the inter-embryo (embryo and surrounding tissues) is required for proliferation, pattern formation, and differentiation during embryogenesis1-3. Moreover, it has long been known that in several plant species, including Arabidopsis, disruption of the original embryonic tissue induces formation of newly embryonic cells derived from the extra-embryonic tissue4-6. However, the process by which an embryonic cell is initiated from the extra-embryonic tissue remains unclear. Angiosperm embryogenesis in vivo occurs within multiple layers of maternal tissues within the flower; therefore it is difficult to observe and analyze the intercellular communications in living material in real time. The development of a live-cell imaging system has been long-awaited to reveal how cell fate is specified by intercellular communication during early embryogenesis. Recently, we have developed a live-embryo imaging system to visualize cell division and cell-fate specification in Arabidopsis thaliana. We incorporated two advanced technologies—micro-engineering and optical manipulation—into this system.
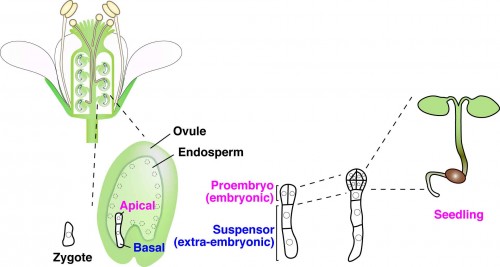
First, we developed an in vitro ovule culture system so that time-lapse imaging could be performed. An in vitro culture system was necessary for real-time observation because embryogenesis could not be observed in vivo on account of the deep tissues. However, we could not observe any cell division in the isolated early embryo7. Therefore, we cultured the embryo within the maternal tissue—the ovules—in vitro. We used liquid medium to prevent the ovules drying up during long-term culture and placed the ovules close to the cover glass. However, stable observation of the ovules in the liquid medium was difficult because of their round shape, so we fabricated PDMS [poly(dimethylsiloxane)] microdevices to adjust the orientation and fix the position of the ovules using micro-engineering techniques.
PDMS has several advantages in the field of developmental biology, such as a high level of biocompatibility, including efficient gas exchangeability and a suitable transparency for observation. PDMS-based microfluidic devices have also been used for animal cell cultures8. Recently, plant researchers have also started to use PDMS-based microdevices for analyzing the growth of root tips, pollen tubes, and seedlings as a lab-on-a-chip9. Initially, we fabricated a PDMS microcage array to fix the ovules in the liquid medium10. To increase the trapping efficiency of the ovules, we modified the PDMS microcage array to a micropillar array11 (Figure 2). Micropillar arrays have a weaker effect on ovule growth because of the physiological flexibility of PDMS micropillars. Thus, we were able to conduct time-lapse imaging of cell division—from the zygote to the heart-stage embryo—for 3 days within the ovules in Arabidopsis embryogenesis.
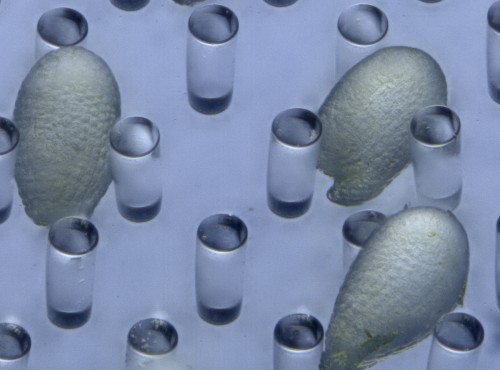
Another advanced technique is optical manipulation. To elucidate the mechanisms of cell–cell communications within the tissues, it is important to carry out a spatio-temporal analysis to determine when, where, and which cells are communicating. Optical manipulation is a powerful tool for such real-time analysis. Because lasers have high optical intensities at the focal point, laser ablation has long been used to disrupt cells. In plants, UV lasers have been used for laser ablation12,13. However, there are limitations to how UV lasers can be used. Short-wavelength lasers, such as UV lasers, are susceptible to refraction, absorption, and scattering from the tissues. Therefore, it is difficult to ablate the cell at the level of a single cell within deep tissues.
Recently, near-infrared pulse lasers have been gradually used to overcome the limitation of UV lasers. Near-infrared pulse lasers are tolerant to scattering within the tissues because of their longer wavelength. In addition, femto-second pulse lasers can induce nonlinear optical effects, such as plasma formation, at a sub-femto-liter volume. Thus it can induce damage even within a cell14. Hasegawa et al. performed the disruption of actin filaments within a tobacco-cultured cell using a femto-second pulse laser in real time15.
We performed laser disruption to our live-embryo imaging system using a femto-second pulse laser11. When an apical cell (embryonic cell) was disrupted by laser irradiation, the cell fate of the remnant basal cell (extra-embryonic cell) was converted, through changes in identity gene expression, into an embryonic cell (Figure 3). In terms of the disruption of basal cells, we found that apical cells were not converted into basal cells. Thus, we succeeded in performing real-time observation of cell-fate conversion for the compensation of an embryonic cell. We propose that the fate and behavior of each individual cell in Arabidopsis embryos is determined via intercellular communication with neighboring cells, and that such flexibility contributes to the robustness of plant embryogenesis.
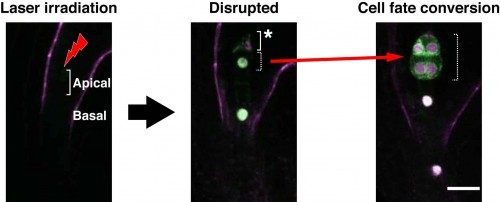
Our imaging system enables direct visualization of the dynamics of cell-fate specification during embryogenesis using micro-engineering techniques. In plants, cell fates are specified via positional information during development. Femto-second laser irradiation, as a form of optical manipulation, enables a spatio-temporal induction of cell-fate conversion that is impossible with genetic ablation. Thus, these techniques will enable novel insights into the molecular mechanisms of cell-fate specification via intercellular communication in plant development. Moreover, these techniques are highly suited to research into developmental biology as well as to plant research, so will be of interest to broad developmental biologists in addition to their applicability to live-cell analysis.
1 Kawashima, T., & Goldberg, R. (2010). The suspensor: not just suspending the embryo Trends in Plant Science, 15 (1), 23-30 DOI: 10.1016/j.tplants.2009.11.002
2 Javelle, M., Vernoud, V., Rogowsky, P., & Ingram, G. (2011). Epidermis: the formation and functions of a fundamental plant tissue New Phytologist, 189 (1), 17-39 DOI: 10.1111/j.1469-8137.2010.03514.x
3 Lafon-Placette, C., & Köhler, C. (2014). Embryo and endosperm, partners in seed development Current Opinion in Plant Biology, 17, 64-69 DOI: 10.1016/j.pbi.2013.11.008
4 Haccius, B. (1955). Experimentally Induced Twinning in Plants. Nature 176, 355-356, doi:Doi 10.1038/176355a0
5 Yeung, E., & Meinke, D. W. (1993). Embryogenesis in Angiosperms: Development of the Suspensor Plant Cell, 5 (10), 1371-1381 DOI: 10.1105/tpc.5.10.1371
6 Schwartz, B. W., Yeung, E. C. & Meinke, D. W. (1994). Disruption of Morphogenesis and Transformation of the Suspensor in Abnormal Suspensor Mutants of Arabidopsis. Development 120, 3235-3245 DOI:
7 Kurihara, D., Hamamura, Y., & Higashiyama, T. (2013). Live-cell analysis of plant reproduction: Live-cell imaging, optical manipulation, and advanced microscopy technologies Development, Growth & Differentiation, 55 (4), 462-473 DOI: 10.1111/dgd.12040
8 Bhatia, S., & Ingber, D. (2014). Microfluidic organs-on-chips Nature Biotechnology, 32 (8), 760-772 DOI: 10.1038/nbt.2989
9 Sanati Nezhad, A. (2014). Microfluidic platforms for plant cells studies Lab on a Chip, 14 (17), 3262-3274 DOI: 10.1039/C4LC00495G
10 Park, J., Kurihara, D., Higashiyama, T., & Arata, H. (2014). Fabrication of microcage arrays to fix plant ovules for long-term live imaging and observation Sensors and Actuators B: Chemical, 191, 178-185 DOI: 10.1016/j.snb.2013.09.060
11 Gooh, K., Ueda, M., Aruga, K., Park, J., Arata, H., Higashiyama, T., & Kurihara, D. (2015). Live-Cell Imaging and Optical Manipulation of Arabidopsis Early Embryogenesis Developmental Cell, 34 (2), 242-251 DOI: 10.1016/j.devcel.2015.06.008
12 van den Berg C, Willemsen V, Hendriks G, Weisbeek P, & Scheres B (1997). Short-range control of cell differentiation in the Arabidopsis root meristem. Nature, 390 (6657), 287-289 PMID: 9384380
13 Higashiyama, T., Yabe, S., Sasaki, N., Nishimura, Y., Kuroiwa, H., & Kuroiwa, T. (2001). Pollen Tube Attraction by the Synergid Cell Science, 293 (5534), 1480-1483 DOI: 10.1126/science.1062429
14 Tirlapur, U., & König, K. (2002). Targeted transfection by femtosecond laser Nature, 418 (6895), 290-291 DOI: 10.1038/418290a
15 Hasegawa, J., Higaki, T., Hamamura, Y., Kurihara, D., Kutsuna, N., Higashiyama, T., Hasezawa, S. & Matsunaga, S. (2014). Vacuole subdivision in plant cell growth by the genotoxic stress inducing DNA double strand breaks. Cytologia 79, 467-474, doi:10.1508/cytologia.79.467