Migratory neuronal progenitors in Tunicates provide insights into Neural Crest evolution
Posted by A. Stolfi, on 24 December 2015
Tunicates are the invertebrates most closely related to us, forming a monophyletic clade with the vertebrates, known as Olfactores. Tunicates, often erroneously referred to as “urochordates” (a junior synonym and thus a taxonomically invalid term), have revealed many insights into the development and evolution of chordate- or vertebrate-specific tissues and organs, such as the notochord, thyroid, liver, craniofacial muscles, heart, spinal cord, and more. Of course, one of the most important vertebrate features is a population of cells called the Neural Crest Cells (NCCs). In all chordates, the central nervous system derives from a dorsal neural plate, which rolls into a dorsal hollow neural tube. In vertebrates, NCCs are specified all along the lateral borders of the neural plate, which meet at the dorsal midline upon neural tube closure. There, NCCs undergo an epithelial-to-mesenchymal transition to form what’s been sometimes called the “Fourth Germ Layer”1. This is because NCCs are a population of stem cell-like progenitors that delaminate and migrate to give rise to a dizzying array of cell types all throughout our bodies and most of the skull: pigment cells, sensory neurons, glia, cartilage, bone, connective tissue, smooth muscle, and chromaffin cells of the adrenal medulla.
Because NCCs are so special to us, there has been much interest in understanding its evolutionary origins. There have been many theories and many disagreements, but a clear picture is starting to emerge. One of the biggest clues pointing to the origin of NCCs is one derivative cell type in particular: pigment cells containing melanin, or melanocytes. In cephalochordates, the other major chordate subphylum, cells along the lateral borders of the neural plate give rise to melanocytes associated with a series of light-sensing organs in the neural tube, known as Dorsal Ocelli2. These are not to be confused with the melanocytes of the frontal eye, which appear to be homologous to the pigment cells of the vertebrate retina instead3. In tunicates, similar dorsal melanocytes arise from the lateral borders of the neural plate and become associated with a light-sensing ocellus and a gravity-sensing otolith4. Recently, the gene regulatory networks specifying and differentiating these cells have been shown to be shared with the NCC-derived melanocytes of vertebrates, strengthening the case for homology5. Therefore, the latest models of NCC evolution propose that the neural plate borders of the pre-vertebrate ancestor already gave rise to one NCC derivative: melanocytes. Presumably, the ability to delaminate, migrate, and differentiate into several different cell types would have been added on to these ancestral melanocyte progenitors6,7.
The major questions stemming from this model are: how did the vaunted multipotency of the NCCs evolve? How did they acquire their amazing migratory capabilities? Were these traits coupled to a singular evolutionary co-option event, or have they been added on, piecemeal, over the course of chordate evolution? To begin answering these questions, one must look carefully at all those other derivatives of vertebrate NCCs Some, like cartilage and bone, are clearly a co-option of an ancestral mesoderm derivative8. Other cell types are likely vertebrate-specific, like chromaffin cells. However, the picture is less clear when looking at NCC-derived neurons, since clear homologs of these have not been found in tunicates or cephalochordates. Although the peripheral nervous systems of tunicate larvae have several sensory neuron subtypes9, none of them have been decisively linked to NCCs, either because they do not arise from the neural plate borders or because they more closely resemble non-NCC-derived sensory cells in vertebrates.
Here I describe and comment on our recent results10, which have further refined our models of NCC evolution. We were intrigued by a particular neuronal subtype that had been recently discovered and described in the tunicate Ciona intestinalis, named the Bipolar Tail Neuron (BTN)9. So called due to their bipolar, or more accurately, pseudo-unipolar morphology, bearing a single axon divided into two long neurites, or processes: an anterior, proximal process extending towards the head of the tadpole, and a posterior, distal process extending to the tip of the tail. We showed that the BTNs receive synaptic inputs from sensory cells embedded in the epidermis of the tail11. Furthermore, we also found that the BTNs synapse onto neurons of the Motor Ganglion, the spinal cord homolog of tunicates. Thus, the BTNs form a relay, part of a neural network that transmits sensory inputs from the periphery to the central nervous system.
This configuration is reminiscent of certain sensory pathways in vertebrates, in which NCC-derived Dorsal Root Ganglia (DRG) neurons relay peripheral sensory inputs from epidermal neurons known as Merkel Cells12, to neurons of the spinal cord and beyond. The shared molecular features are also intriguing, as both DRG neurons and BTNs express the transcription factor genes Neurogenin (Neurog) and Islet 13-15, and mechanosensation-modulating Acid-Sensing Ion Channels (ASICs)16. These parallels prompted us to wonder whether the BTNs, like the melanocytes of Ciona, could represent an evolutionary forebear of the NCCs. We reasoned that further support for this possibility would come from identifying the embryological origins of the BTNs (whether they originate at the borders of the neural plate), and characterizing their development (whether they engage in delamination and migration).
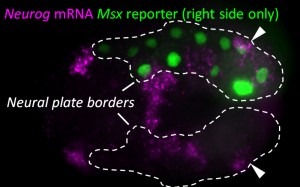
Since we knew that the BTNs express Neurog, we looked at the expression of this gene by mRNA in situ hybridization, and found that it is activated in two lateral rows of cells at the very posterior end of the embryo at the early neurula stage (Figure 1). We showed that these cells are at the boundary between the epidermis and neural tissue, and express Msx and Pax3/7, conserved markers of the neural plate borders in all chordates. In vertebrates, NCCs arise from Msx+/Pax3/7+ neural plate border cells, and in fact these genes encode upstream regulators of many NCC specification genes17. Using a Neurog reporter gene to follow these cells throughout development, we found that BTN precursors delaminate shortly after neural tube closure, and migrate as a simple chain of two cells on either side of the neural tube (Figure 2). We found that they migrate outside the neural tube, along paraxial mesoderm, evocative of the migration of NCC-derived DRG neuroblasts along paraxial mesoderm on either side of the vertebrate neural tube. As the BTNs migrate anteriorly to the middle of the tail, their intrinsic polarity is reversed and begin to extend their posterior processes, suggesting a precisely timed re-orientation of cell polarity underlies their distinctive bipolar morphology.
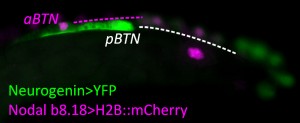
We also investigated the molecular control of BTN specification and differentiation, and found that this is under the control of Neurog itself, which in turn is tightly regulated by FGF/ERK signaling. While we are still working to sort out these steps in detail, our current findings as they stand have some interesting implications for models of NCC evolution. Mainly, we present evidence that the BTNs and the DRG neurons might be homologous, which would imply that last common ancestor of vertebrates and tunicates might have had neural plate borders capable of giving rise to more than one NCC derivative cell type, in this case, pigment cells and sensory relay neurons. Furthermore, our observation of the delamination and migration of the BTNs along the paraxial mesoderm suggests that some aspects of NCC migratory behavior may also pre-date the emergence of vertebrates and bona fide NCCs.
Our new model, building on previous models, posits that the olfactorean ancestor already had neural plate borders capable of generating both pigment cells and sensory relay neurons, which in vertebrates are two derivatives of NCCs. In the highly reduced tunicate embryo, pigment cells and sensory neurons arise from opposite ends of the neural plate borders. However, recall that cephalochordates have serial pigmented dorsal ocelli, so perhaps the original chordate ancestor generated multiple pigment cell and sensory neurons all along the neural plate borders. In this model the real novelty of vertebrate NCCs is not the ability to give rise to multiple cell types, which in our model is an ancestral character. Instead, the key difference would have been the intercalation of a multipotent stem cell-like regulatory program within the ancestral network, downstream of neural plate border specification and upstream of multiple cell type differentiation programs18. This multipotent progenitor state, a hallmark of NCCs, allowed for these cells to undergo a prolonged period of delamination, migration, proliferation, and more dynamic modes of downstream cell fate specification and differentiation. In sum, our findings lend further support to the longstanding idea that vertebrate NCCs were not an abrupt vertebrate innovation, but rather derived from a set of ancestral features, elaborated over the course of chordate evolution19.
1 Hall, B. K. The neural crest as a fourth germ layer and vertebrates as quadroblastic not triploblastic. Evolution & development, 3-5 (2000).
2 Yu, J.-K., Meulemans, D., McKeown, S. J. & Bronner-Fraser, M. Insights from the amphioxus genome on the origin of vertebrate neural crest. Genome research 18, 1127-1132 (2008).
3 Vopalensky, P. et al. Molecular analysis of the amphioxus frontal eye unravels the evolutionary origin of the retina and pigment cells of the vertebrate eye. Proceedings of the National Academy of Sciences 109, 15383-15388 (2012).
4 Nishida, H. & Satoh, N. Determination and regulation in the pigment cell lineage of the ascidian embryo. Developmental Biology 132, 355-367 (1989).
5 Abitua, P. B., Wagner, E., Navarrete, I. A. & Levine, M. Identification of a rudimentary neural crest in a non-vertebrate chordate. Nature 492, 104-107 (2012).
6 Wada, H. Origin and evolution of the neural crest: a hypothetical reconstruction of its evolutionary history. Development, growth & differentiation 43, 509-520 (2001).
7 Ivashkin, E. & Adameyko, I. Progenitors of the protochordate ocellus as an evolutionary origin of the neural crest. EvoDevo 4, 12 (2013).
8 Jandzik, D. et al. Evolution of the new vertebrate head by co-option of an ancient chordate skeletal tissue. Nature 518, 534-537 (2015).
9 Imai, J. H. & Meinertzhagen, I. A. Neurons of the ascidian larval nervous system in Ciona intestinalis: II. Peripheral nervous system. The Journal of comparative neurology 501, 335-352 (2007).
10 Stolfi, A., Ryan, K., Meinertzhagen, I. A. & Christiaen, L. Migratory neuronal progenitors arise from the neural plate borders in tunicates. Nature 527, 371-374 (2015).
11 Torrence, S. A. & Cloney, R. A. Nervous system of ascidian larvae: caudal primary sensory neurons. Zoomorphology 99, 103-115 (1982).
12 Maksimovic, S. et al. Epidermal Merkel cells are mechanosensory cells that tune mammalian touch receptors. Nature 509, 617-621 (2014).
13 Ma, Q., Fode, C., Guillemot, F. & Anderson, D. J. Neurogenin1 and neurogenin2 control two distinct waves of neurogenesis in developing dorsal root ganglia. Genes & development 13, 1717-1728 (1999).
14 Sun, Y. et al. A central role for Islet1 in sensory neuron development linking sensory and spinal gene regulatory programs. Nature neuroscience 11, 1283-1293 (2008).
15 Stolfi, A. & Christiaen, L. Genetic and genomic toolbox of the chordate Ciona intestinalis. Genetics 192, 55-66 (2012).
16 Coric, T., Passamaneck, Y. J., Zhang, P., Di Gregorio, A. & Canessa, C. M. Simple chordates exhibit a proton-independent function of acid-sensing ion channels. The FASEB Journal 22, 1914-1923 (2008).
17 Simões-Costa, M. & Bronner, M. E. Establishing neural crest identity: a gene regulatory recipe. Development 142, 242-257 (2015).
18 Bronner, M. E. & LeDouarin, N. M. Development and evolution of the neural crest: an overview. Developmental biology 366, 2-9 (2012).
19 Baker, C. V. & Bronner-Fraser, M. The origins of the neural crest. Part II: an evolutionary perspective. Mechanisms of development 69, 13-29 (1997).