The dorsal root of the matter: Using zebrafish to study the importance of movement on early brain growth
Posted by Zach Hall, on 8 May 2018
In our recent paper published in eLife, we found a novel form of movement-dependent neural feedback that drives early forebrain growth in zebrafish. In this article, I discuss the problems, solutions, and lucky breaks that led to our finding. I also end up giving the mighty zebrafish larvae the credit it so deserves.
A year before the completion of my PhD, I unofficially joined Vince Tropepe’s lab at the University of Toronto as a postdoc over the course of a two hour Skype call. During this call, we slowly came to recognize one another as our academic complements: Vince’s history is firmly rooted in one end of the organismal spectrum, the genetics and cell biology of stem cells, extending up to brief forays into how sensory experiences modulate adult neurogenesis in vertebrates. Conversely, with no history in genetics or cell biology, I had started at the other end of the organismal spectrum, studying the evolution of brain structure and animal behaviour and extending down to how sensory experiences modulate adult neurogenesis in vertebrates. With a common interest in how the environment shapes brain development, together we felt that our collective expertise might offer a novel perspective to studying the importance of sensory experience on the production of new brain cells. Also, Vince promised me that I would become a competent geneticist–a promise we are still both working on today.
A year later, I arrived at the University of Toronto and met both Vince and zebrafish. The fish were much smaller than in the figures. Eager to delve into the behavioural repertoire of zebrafish, I purchased my own set of adults from a local pet store and watched them daily from home. Whereas my mind was dancing among work documenting the acoustic communications of midshipman fish, territoriality in cichlids, and nest construction in sticklebacks, my eyes were settled on a set of four fish that seemed to simply swim and eat and swim again. The fish neither sang nor built a nest, two behaviours I had previously studied in songbirds throughout grad school. Why were people studying zebrafish again?
In countless reviews, I had read that the power of studying zebrafish is in the accessibility of the embryo during development and their genetic tractability. The former of these advantages is evident during the first 24 hours of zebrafish development, over which one can watch a pigment-less embryo develop into a larvae in real time. Now one day old, the genetic tractability of zebrafish became obvious in the variety of transgenic zebrafish strains available in Vince’s lab, starting to glow red or green or red-and-then-green beneath the stereomicroscope. While I may have been taking the nicest micrographs of my career, I soon became worried about my choice in model system if all of the advantages of working with zebrafish–transparent embryos; the ability to introduce mutations or transgenes–only applied to the first couple days of development. At these early stages, larvae had yet to develop the neural connections to process sensory information and by the time they did, pigment would (rudely) form in the skin, blocking my view of the brain. I feared that I was trying to drag zebrafish larvae out of the developmental window for which they were chosen as a model system.
As I assume many postdocs do, I started in the lab bursting with creative energy and began experimenting by instead repeating a previous lab member’s work only with a slightly different focus. In my case, I was building off of original work by Ben Lindsey, who had shown in a collection of studies how visual, social, and olfactory experiences modulate the production and survival of new neurons in the adult zebrafish brain (Lindsey and Tropepe, 2014; Lindsey et al., 2014). Ben had integrated zebrafish into the popular field of experience-dependent adult neurogenesis, dominated by rodent models but also involving a menagerie of other vertebrates including amphibians, lizards, fish, and birds. My work differed from Ben’s in that I was manipulating sensory experience in zebrafish larvae only days old, compared to the adult fish in his previous studies. The decision to study postembryonic brain development instead of adult neurogenesis was motivated by both practical advantages and, more importantly, theoretical reasons. Practically, larvae are tiny and easy to manipulate: we could generate hundreds of larvae and even still reap some of the advantages of transgenic strains, such as fluorescent labeling of neuronal populations in histological sections. Theoretically, Vince and I had come to question whether adulthood was the developmental stage best suited to our questions.
Most often, the study of neurogenesis in the vertebrate brain is experimentally bimodal: studies focus on either embryonic neurogenesis, in which neurons are generated to initially produce a central nervous system, or adult neurogenesis, where the brain is functionally mature and neurons continue to be added at the relatively lowest rate in life. We found ourselves interested in the awkward developmental period in between these extremes, broadly referred to here as postembryonic development. Somewhere in postembryonic development, the brain must become sufficiently developed to begin processing incoming sensory input from the environment. During this time, the brain is also still growing, including the incorporation of new neurons. The combination of sensory processing and continued brain growth make postembryonic development the period of brain growth most sensitive to sensory experience. This postembryonic sensitivity is thought to explain why learning new languages or musical instruments is easier earlier than later in life (White et al., 2013). Within the nervous system, these forms of experience-dependent neuroplasticity are traditionally considered to occur through modifications in the connections between pre-existing neurons. We asked whether the continued production of new neurons itself may also mediate the effects of experience on early brain growth.
With a focus on postembryonic neurogenesis, I began a multitude of experiments manipulating the sensory environments of zebrafish larvae. Initially, my work focused on modifying the visual experiences of larvae by rearing them in different lighting conditions and tracking the developmental trajectory of both the retina and its primary target in the midbrain, the optic tectum. This approach benefitted from the extensive published work characterizing 1) neural circuitry in the retinotectal pathway of zebrafish, 2) the switch from spontaneous to visual input-dependent neural activity in the optic tectum upon maturation of visual input by 5 days of age, and 3) Ben’s previous work demonstrating that visual experience would modulate neurogenesis in zebrafish. These experiments culminated in a publication released earlier this year in the Journal of Neuroscience (Hall and Tropepe, 2018a), in which we discovered that visual experience-dependent growth of the zebrafish midbrain is mediated, at least in part, by the modulation of new neuron survival.
Parallel with my work on visual experience, I was also piloting approaches to noninvasively manipulate social and olfactory experience in zebrafish larvae. Across all of these studies, I discovered a novel experimental advantage in using zebrafish that I had never seen reported in a literature review. It turns out that zebrafish larvae are amenable to extreme manipulations in the sensory environment. This advantage is conferred by the combination of the zebrafish larva’s small size, built-in food source (a yolk providing nutrition for the zebrafish for up to 5-6 days of age), and easily met living conditions. Using zebrafish larvae, I was able to isolate, restrict, and enrich distinct sensory modalities noninvasively without grossly altering the larva’s ability to develop. Perhaps the best example of this sensory tractability I’m referring to is in our recently published paper in eLife (Hall and Tropepe, 2018b).
My work on motor experience began after I stumbled upon the ViewPoint Zebrabox, a chamber designed to track zebrafish larval swimming behaviour, sitting idly in one of my testing rooms. Vince explained that he had purchased the system using an equipment grant years ago after a previous postdoc, Bruno Souza, had the unfortunate opportunity to manually code larval swimming from video. Bruno discovered that disruptions to dopamine signaling during postembryonic brain development reduced both the size of an inhibitory neuron population in the zebrafish forebrain and the amount a fish would swim (Souza et al., 2011). One conclusion from this work was that perhaps this inhibitory neuron population is required to support normal motor development. Looking to be a contrarian, I argued the opposite, that the amount a fish swims may guide the development of the neuronal population. Intent on settling the issue, we brainstormed techniques through which we could transform movement, typically recorded as a dependent variable, into a manipulation itself. Since the discovery that aerobic exercise upregulates adult neurogenesis in mammals (van Praag et al., 1999), movement has overwhelmingly been manipulated positively with the use of stationary wheels and wind/water tunnels to encourage running, swimming, and flying. Still a contrarian, I suggested we instead restrict movement, restraining larvae within small cylindrical mesh fences to reduce the available swim space in a similar volume of water compared to controls.
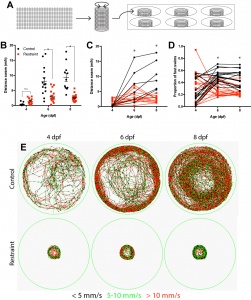
One summer semester later, and with the help of one eager undergraduate student, I had my answer as to whether movement drives the development of this inhibitory neuronal population: no. Although our restriction paradigm significantly reduced swimming from both 3-6 and 3-9 days, we could account for every inhibitory neuron produced regardless of swimming experience. Luckily, we noticed something else. When we restrained larval swimming for as long as 6 days, the entire inhibitory neuronal population was there, sure, but the forebrain itself was significantly smaller in our restrained larvae, suggesting that movement could be modulating neurogenesis, albeit it not via the production of the neurons we were focusing on.
Using a variety of histological markers and changing our focus from the neurons being produced to the cells producing them, the neural stem cell and intermediate progenitor populations, we found that restricting swimming led to fewer proliferative cells in the dorsal portion of the forebrain, referred to as the pallium. Subsequent analysis found that this reduction was not attributed to cell death or a change in the population of neural stem cells, but a reduction in the size of the intermediate progenitor populations, which amplify the rate of neurogenesis by adding additional proliferative steps between stem cell and differentiated neuron. Recognizing that reducing swimming by rearing larvae in less physical space may be complicated by reductions in other sensory inputs, such as reduced visual input with less to see, we complemented our approach by rearing larvae against water currents to increase swimming. Using this approach, we conversely found that increasing swimming also increased the population of proliferative cells in the pallium. Together, these experiments formed the basis for our mechanistic work probing how movement itself modulates early brain development.
Thus far, our work seemed to fit nicely among the extensive literature on exercise-dependent increases in vertebrate adult neurogenesis, with the added benefit that we could manipulate movement in both directions. Having learned that working in a cellular and molecular biology department meant you should draw a lot of mechanistic flowcharts, I took a stab at it.
Though wildly underwhelming, the simple framing of movement as affecting neurogenesis led us to discover a notable gap in the literature. Mechanistic work on exercise-dependent adult neurogenesis has implicated genes, neurotransmitters, and growth factors that act within the neurogenic niche. However, these proliferative populations do not often receive direct input from the body, leaving the question as to how the body signals the brain to increase neuron production. Current theories are centred around the concept that muscle engagement could release trophic factors into circulation that are carried to the brain to stimulate growth, though this model is difficult to test. We argued that the best step towards linking bodily movement to brain growth was to ask, “what sort of information does movement provide the brain?” and how each of these modalities may be isolated or removed during swimming. Enter the tolerant zebrafish larvae.
Optic flow is the term referring to the visual illusion of movement. As we walk forward, the visual environment appears to move backward. This visual input is processed by the brain to maintain balance during movement and explains why, when stopped in traffic, a neighbouring cars rolling forward creates the illusion that we are rolling backward. In our first experiment, we aimed to divide swimming into a visual component and a physical component. But how could we trick a fish that isn’t swimming into perceiving movement? And conversely, how could we allow a fish to swim without moving throughout the environment? Our solution came from a common treatment in many zebrafish imaging studies–agarose embedding. Although immobilizing larvae in agarose is commonly used in calcium imaging or electrophysiological preparations, these preparations are relatively acute. We found that we could chronically immobilize larvae using a sufficiently low concentration of agarose gel. Embedded larvae are unable to move but remain alive via water flow through agarose and by feeding on the yolk they are born with. Unable to move, we then reintroduced sensory components of movement to our fish. Optic flow was simulated by exposing animals to computerized black and white gratings moving in a given direction. Free-swimming larvae will swim parallel to the direction of movement of a grating (perpendicular to the stripes of the grating). Exposing larvae to a battery of moving gratings through days 3-6 acted as a form of visual movement-dependent input. To reintroduce physical components of movement, we cut larval tails free from the agarose, enabling the bodily movement of swimming without physical displacement in the environment. Using these manipulations, we found that only physical input associated with swimming (tail movement) stimulated increased neurogenesis in the larval forebrain, leading us to cross visual input off the list.
Unlike visual input, which would predictably originate from the retina, physical input during swimming could be sensed by multiple systems in larval zebrafish. Externally, the movement of water against the skin could be detected by the lateral line, a system of cell clusters along the length of teleost fish containing hair cells that extend their cilia outside of the body into the external environment. Water movement causes the cilia in hair cells to bend, which then drives ascending input to the central nervous system. Because of the exposed nature of these hair cells, they can be easily ablated through the treatment of chemicals added to the fish water. We found that ablating lateral line hair cells had no impact on forebrain neurogenesis, suggesting this pathway was not mediating the effects of movement on brain growth observed here.
Internally, bodily movement in zebrafish larvae could be detected via two neuronal populations throughout the trunk of the fish.
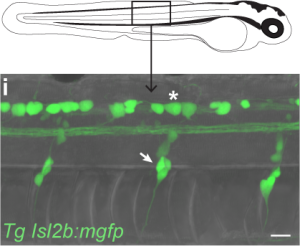
The first population, Rohon-Beard cells, lie within the spine and extend somatosensory processes throughout the skin. This population of neurons develop early in zebrafish development and appear to die off entirely by adulthood. The second population, dorsal root ganglia, lie on each side of the spine and first appear only by 3 days of age, but will exist for the rest of the fish’s life. Upon identifying these neuronal populations, we at first rejoiced at the opportunity to take advantage of the transgenic tools available in zebrafish, isolating these populations by the genes they uniquely express. While searching for transgenic lines reported to include targeting to either Rohon-Beard cells or dorsal root ganglia, I stumbled upon a bit of luck. One of the transgenic lines isolating Rohon-Beard cells under the expression of the isl2b promoter was already in the lab! Vince had received this line from Chi Bin Chien and I had been using it for the past year as this promoter also isolates the projection from the fish retina to the optic tectum! I reared a clutch of larvae from this transgenic line and inspected their bodies to find bright green Rohon-Beard cells! Unfortunately, I also found bright green dorsal root ganglia beside them. Expression of our fluorescent reporter in both cell populations meant we would be unable to isolate these different sensory inputs genetically.
With a little luck and I lot of reading, I soon found another approach to isolating Rohon-Beard cells and dorsal root ganglia in the work of Judith Eisen’s lab. The Eisen lab had found that precursor cells that would ultimately give rise to dorsal root ganglia must first migrate out of the spine into the body. These precursors require a specific cell signal to tell them to stop in the correct location to generate dorsal root ganglia. If this signal is blocked during this migration time using the drug AG1478, the precursors continue to migrate aberrantly, failing to give rise to any dorsal roots (Honjo et al., 2008). Replicating these results in the lab, we found that acute treatment with low doses of AG1478 blocked the initial formation of dorsal root ganglia, generating larvae lacking one of these two neuronal populations. With a batch of zebrafish larvae lacking a dorsal root ganglia population in the trunk, we repeated our swimming restraint experiments. We found that larvae deficient in dorsal root ganglia exhibit attenuated swimming-dependent forebrain neurogenesis, suggesting dorsal roots mediate sensory feedback during swimming to maintain an expanded pool of proliferating cells in the forebrain.
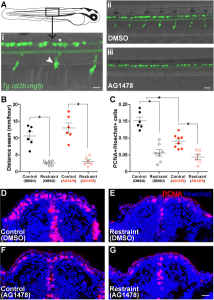
At this point, we were beginning to feel confident that we may have identified one form of sensory feedback during movement that could drive forebrain growth. And unlike previous models, which suggested these signals would be transported via the circulatory system, our results suggested this input could also come directly from peripheral nervous input. However, like our manipulations of swimming behaviour in the original experiments, we believed that removing dorsal root ganglia and finding a reduction in neurogenesis should be complemented with an experiment in which we drive neurogenesis using peripheral neural input, aimed primarily at dorsal root ganglia. Based on our experiments up to this point, we predicted that activating this peripheral sensory input could drive increased forebrain neurogenesis, even in the complete absence of movement.
After a handful of failed pilots in which I had tested drugs I was hoping may enhance neuronal firing in dorsal root ganglia, I met with Vince, worried our study may have to stop one experiment short of our plans. I was unable to believe there was not some known drug that could be added to the water to activate dorsal root ganglia in zebrafish. Vince was also unable to believe this, particularly after just having sat through a PhD committee meeting earlier that day in which he listened to a graduate student report on Optovin, a drug characterized by Randy Peterson’s lab that can be added to the water to activate peripheral neural feedback, including dorsal root ganglia. Eureka (I should have said). Optovin is a small molecule that interacts with the TRPA1b receptor found in the peripheral nervous system of zebrafish, changing the dynamics of these receptors to open following exposure to purple light (Kokel et al., 2013). With this stroke of luck and a batch of Optovin aliquots generously provided by Jim Dowling, we were able to control peripheral nervous feedback in larval zebrafish embedded in agarose, unable to move. Furthermore, we found that a single session of light pulses in Optovin-treated larvae produced a significant increase in the number of proliferating cells in the zebrafish pallium as early as the next morning. When we repeated these light + Optovin treatments in larvae deficient in dorsal root ganglia, the effect was blocked, consistent with a direct role for dorsal root ganglia in providing neural feedback during swimming to encourage forebrain growth via increased neurogenesis.
Altogether, we believe our study provides a novel perspective on the importance of movement in early brain development both because of our model of sensory feedback, but also for the age of the fish we study. First, our findings suggest a wholly novel form a sensory feedback during movement that may act as a means of coordinating body and brain development. Fish that swam the most also exhibited the highest rates of neurogenesis. Second, although we have borrowed much of our theory from the study of exercise-dependent neurogenesis, we believe our results here may be unique in the examination of postembryonic brain development. Often considered simply an early form of the slower neurogenesis persisting into adulthood, increasing work has suggested that postembryonic neurogenesis in developmentally unique, producing populations of neurons not generated elsewhere in life. Accordingly, we believe our work may specifically highlight the importance of movement-generated sensory feedback during early brain growth in vertebrates. Such a coordination between brain and behaviour may explain the comorbidity of cognitive deficits in diseases predominantly considered to be muscular, such as congenital myopathy.
As a study, we believe the power in our results comes from the collection of experiments we performed as a whole. Whereas we often performed experiments that were met with technical limitations, we could always generate a complementary experiment to overcome these restrictions. And all along the way, from mesh fences to swim tunnels to immobilization and Optovin jolts, we believe no other model organism would’ve kept on developing as well as the zebrafish larvae. So what if they don’t build a nest?
References
- Hall, Z. J., & Tropepe, V. (2018a). Visual experience facilitates BDNF-dependent adaptive recruitment of new neurons in the postembryonic optic tectum. Journal of Neuroscience, 38(8), 2000-2014.
- Hall, Z. J., & Tropepe, V. (2018b). Movement maintains forebrain neurogenesis via peripheral neural feedback in larval zebrafish. eLife, 7, e31045.
- Honjo, Y., Kniss, J., & Eisen, J. S. (2008). Neuregulin-mediated ErbB3 signaling is required for formation of zebrafish dorsal root ganglion neurons. Development, 135(15), 2615-2625.
- Kokel, D., Cheung, C. Y. J., Mills, R., Coutinho-Budd, J., Huang, L., Setola, V., … & Bruni, G. (2013). Photochemical activation of TRPA1 channels in neurons and animals. Nature chemical biology, 9(4), 257.
- Lindsey, B. W., Di Donato, S., Kaslin, J., & Tropepe, V. (2014). Sensory‐specific modulation of adult neurogenesis in sensory structures is associated with the type of stem cell present in the neurogenic niche of the zebrafish brain. European Journal of Neuroscience, 40(11), 3591-3607.
- Lindsey, B. W., & Tropepe, V. (2014). Changes in the social environment induce neurogenic plasticity predominantly in niches residing in sensory structures of the zebrafish brain independently of cortisol levels. Developmental neurobiology, 74(11), 1053-1077.
- Souza, B. R., Romano-Silva, M. A., & Tropepe, V. (2011). Dopamine D2 receptor activity modulates Akt signaling and alters GABAergic neuron development and motor behavior in zebrafish larvae. Journal of Neuroscience, 31(14), 5512-5525.
- Van Praag, H., Kempermann, G., & Gage, F. H. (1999). Running increases cell proliferation and neurogenesis in the adult mouse dentate gyrus. Nature neuroscience, 2(3), 266.
- White, E. J., Hutka, S. A., Williams, L. J., & Moreno, S. (2013). Learning, neural plasticity and sensitive periods: implications for language acquisition, music training and transfer across the lifespan. Frontiers in systems neuroscience, 7, 90.