January in preprints
Posted by the Node, on 5 February 2025
Welcome to our monthly trawl for developmental and stem cell biology (and related) preprints.
The preprints this month are hosted on bioRxiv – use these links below to get to the section you want:
- Patterning & signalling
- Morphogenesis & mechanics
- Genes & genomes
- Stem cells, regeneration & disease modelling
- Plant development
- Environment, evolution and development
Congratulations to everyone involved in the preprints! As usual, let us know if we’ve missed anything in this list.
Developmental biology
| Patterning & signalling
Luuli N. Tran, Ashwini Shinde, Kristen H. Schuster, Aiman Sabaawy, Emily Dale, Madalynn J. Welch, Trevor J. Isner, Sylvia A. Nunez, Fernando García-Moreno, Charles G. Sagerström, Bruce H. Appel, Santos J. Franco
Robert D Morabito, David Tatarakis, Ryan Swick, Samantha Stettnisch, Thomas F Schilling, Julia A Horsfield, Benjamin L Martin
Gokcen Gozum, Lisa Wirtz, Mareike Damen, Viktoria Reckert, Peter Schettina, Melanie Nelles, Hisham Bazzi, Catherin Niemann
Cell heterogeneity and fate bistability drive tissue patterning during intestinal regeneration
C. Schwayer, S. Barbiero, D. B. Brückner, C. Baader, N. A. Repina, O. E. Diaz, L. Challet Meylan, V. Kalck, S. Suppinger, Q. Yang, J. Schnabl, U. Kilik, J. G. Camp, B. Stockinger, M. Bühler, M. B. Stadler, E. Hannezo, P. Liberali
Ezra E. Amiri, Ayse Tenger-Trolander, Muzi Li, Alexander Thomas Julian, Koray Kasan, Sheri A. Sanders, Shelby Blythe, Urs Schmidt-Ott
Juan Manuel García-Arias, Gonzalo G. Girón, David Foronda, Isabel Guerrero, Antonio Baonza
Endothelial Slit2 guides the Robo1-positive sympathetic innervation during heart development
Juanjuan Zhao, Susann Bruche, Konstantinos Lekkos, Carolyn Carr, Joaquim Miguel Vieira, John Parnavelas, William D Andrews, Mathilda Mommersteeg
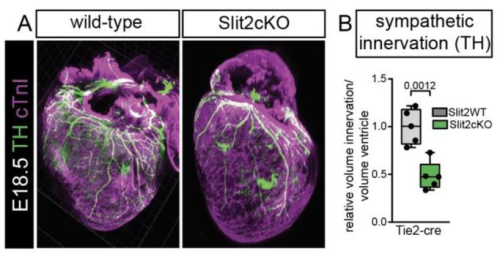
André Dias, Pau Pascual-Mas, Gabriel Torregrosa-Cortés, Harold M. McNamara, Alexandra E. Wehmeyer, Sebastian J. Arnold, Alfonso Martinez Arias
Scaling of mouse somitogenesis by coupling of cell cycle to segmentation clock oscillations
Marek J. van Oostrom, Yuting I. Li, Wilke H. M. Meijer, Tomas E. J. C. Noordzij, Charis Fountas, Erika Timmers, Jeroen Korving, Wouter M. Thomas, Benjamin D. Simons, Katharina F. Sonnen
Sandy Nandagopal, Anna Cha, Bill Z. Jia, Hongyu Liao, Caroline Comenho, Galit Lahav, Daniel E. Wagner, Tony Y-C Tsai, Sean G. Megason
Max Luf, Priya Begani, Anne M. Bowcock, Cathie M. Pfleger
Hyung-seok Kim, Mary L. Sanchez, Joshua Silva, Heidi L. Schubert, Rebecca Dennis, Christopher P. Hill, Jan L. Christian
Receptor Allostery Promotes Context-Dependent Sonic Hedgehog Signaling During Embryonic Development
Shariq S. Ansari, Miriam E. Dillard, Mohamed Ghonim, Yan Zhang, Daniel P. Stewart, Robin Canac, Ivan P. Moskowitz, William C. Wright, Christina A. Daly, Shondra M. Pruett-Miller, Jeffrey Steinberg, Yong-Dong Wang, Taosheng Chen, Paul G. Thomas, James P. Bridges, Stacey K. Ogden
Ana Cristina Ojalvo-Sanz, María Figueres-Oñate, Sonsoles Barriola, Carolina Pernia-Solanilla, Rebeca Sanchez-Gonzalez, Lina Delgado García, Laura López-Mascaraque
Naomi E. Butler Tjaden, Stephen R. Shannon, Christopher W. Seidel, Melissa Childers, Kazushi Aoto, Lisa L. Sandell, Paul A. Trainor
Yingying Chen, Fengxiang Tan, Qing Fang, Lin Zhang, Jiaoyang Liao, Penglei Shen, Yun Qian, Mingzhu Wen, Rui Song, Yonggao Fu, He Jax Xu, Ran Wang, Cheng Li, Zhen Shao, Jinsong Li, Naihe Jing, Xianfa Yang
| Morphogenesis & mechanics
Iona G. Thelwall, Carola M. Morell, Dominika Dziedzicka, Lucia Cabriales, Andrew Hodgson, Floris J.M. Roos, Louis Elfari, Ludovic Vallier, Kevin J. Chalut
Eunah Chung, Fariba Nosrati, Mike Adam, Andrew Potter, Mohammed Sayed, Benjamin D. Humphreys, Hee-Woong Lim, Yueh-Chiang Hu, S. Steve Potter, Joo-Seop Park
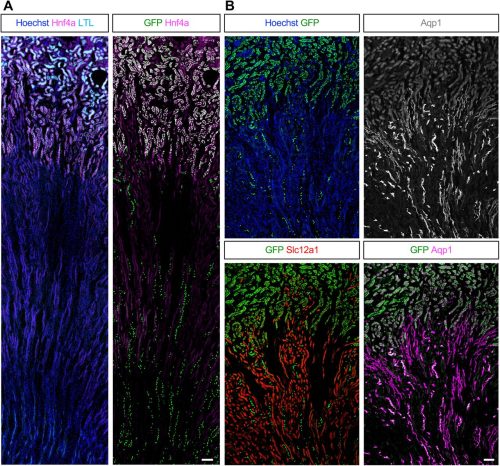
Extracellular stiffness regulates site-specific lung development
Zhiying Liao, Junjie Lv, Dong Wang, Xuepeng Chen, Jincun Zhao, Tao Xu, Hao Meng, Huisheng Liu
Resolving forebrain developmental organisation by analysis of differential growth patterns
Elizabeth Manning, Kavitha Chinnaiya, Caitlyn Furley, Dong Won Kim, Seth Blackshaw, Marysia Placzek, Elsie Place
Kouhei Toga, Kakeru Yokoi, Toru Togawa
Khaoula El Marzkioui, Isabelle Gaugué, Ettore De Giorgio, Pierre Léopold, Laura Boulan
Dena M. Leerberg, Gabriel B. Avillion, Rashmi Priya, Didier Y.R. Stainier, Deborah Yelon
Drosophila Toll-2 controls homeotic domain formation and timing of folding
Lale Alpar, Rémi Pigache, Adrien Leroy, Mehdi Ech-Chouini, Stéphane Pelletier, Yohanns Bellaïche
Non-invasive characterization of oocyte deformability in micro-constrictions
Lucie Barbier, Rose Bulteau, Behnam Rezaei, Thomas panier, Elsa Labrune, Marie-Hélène Verlhac, Franck Vernerey, Clement Campillo, Marie-Emilie Terret
Fine-tuning mechanical constraints uncouple patterning and gene expression in murine pseudo-embryos
Judith Pineau, Jerome Wong-Ng, Alexandre Mayran, Lucille Lopez-Delisle, Pierre Osteil, Armin Shoushtarizadeh, Denis Duboule, Samy Gobaa, Thomas Gregor
Vanessa Weichselberger, Ramya Balaji, Marta Rodriguez-Franco, Anne-Kathrin Classen
Convergent flow-mediated mesenchymal force drives embryonic foregut constriction and splitting
Rui Yan, Ludwig A. Hoffmann, Panagiotis Oikonomou, Deng Li, ChangHee Lee, Hasreet Gill, Alessandro Mongera, Nandan L. Nerurkar, L. Mahadevan, Clifford J. Tabin
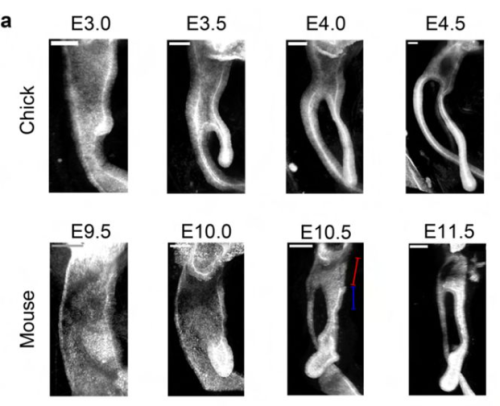
| Genes & genomes
LIN28-mediated gene regulatory loops synchronize developmental transitions throughout organogenesis
Indhujah Thevarajan, Maria F. Osuna, Sonia Fuentes Lewey, Eustolia Sauceda, Sayra Briseno, Caylah Griffin, Bareun Kim, R. Grant Rowe, Edroaldo Lummertz da Rocha, Jihan K Osborne
Identification of Specialized tRNA Expression in Early Human Brain Development
Alex L Bagi, Todd M Lowe, Sofie R Salama
Meghana S. Oak, Marco Stock, Matthias Mezes, Tobias Straub, Antony M. Hynes-Allen, Jelle van den Ameele, Ignasi Forne, Andreas Ettinger, Axel Imhof, Antonio Scialdone, Eva Hörmanseder
p16.1 and p16.2, new HSPC markers, play redundant roles in zebrafish T-cell lymphopoiesisv
Etienne Gomez, Roman A. Li, Julien Y. Bertrand
Gist H. Farr III, Whitaker Reid, Isabelle Young, Mona L. Li, David R. Beier, Lisa Maves
Angel Tevar, Jose-Daniel Aroca-Aguilar, Raquel Atiénzar-Aroca, Ana I. Ramírez, José A. Fernández-Albarral, Julio Escribano
Gyunghee G. Lee, Aidan J. Peterson, Myung-Jun Kim, MaryJane Shimell, Michael B. O’Connor, Jae H. Park
Rekha M. Dhillon-Richardson, Alexandra K. Haugan, Luke W. Lyons, Joseph K. McKenna, Marianne E. Bronner, Megan L. Martik
LINE-1 retrotransposons regulate the exit of human pluripotency and early brain development
Anita Adami, Raquel Garza, Patricia Gerdes, Pia A. Johansson, Fereshteh Dorazehi, Symela Koutounidou, Laura Castilla-Vallmanya, Diahann A.M. Atacho, Yogita Sharma, Jenny G. Johansson, Oliver Tam, Agnete Kirkeby, Roger A. Barker, Molly Gale-Hammell, Christopher H. Douse, Johan Jakobsson
Chd4 remodels chromatin to control retinal cell type specification and lineage termination
Sujay Shah, José Alex Lourenço Fernandes, Suma Medisetti, Pierre Mattar
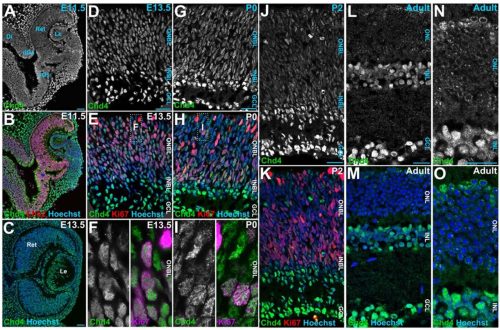
So Maezawa, Masashi Yukawa, Akihiko Sakashita, Artem Barski, Satoshi H. Namekawa
Nancy Ashary, Sanjana Suresh, Anshul Bhide, Sharmishtha Shyamal, N Pranya, Anuradha Mishra, A Anuradha, Shruti Hansda, B V Harshavardhan, Mohit Kumar Jolly, Deepak Modi
Foxi2 and Sox3 are master regulators controlling ectoderm germ layer specification
Clark L. Hendrickson, Ira L. Blitz, Amina Hussein, Kitt D. Paraiso, Jin Cho, Michael W. Klymkowsky, Matthew J. Kofron, Ken W.Y. Cho
Simon Desiderio, Pauline Cabochette, Stephanie Venteo, Gautier Tejedor, Farida Djouad, Patrick Carroll, Fabrice Ango, Alexandre Pattyn
Ana Bermejo-Santos, Rodrigo Torrillas-de la Cal, Miguel Rubio-García, Esther Serrano-Saiz
Transcriptional Integration of Meiotic Prophase I Progression and Early Oocyte Differentiation
Kimberly M. Abt, Myles A. Bartholomew, Anna Nixon, Hanna E. Richman, Megan A. Gura, Kimberly A. Seymour, Richard N. Freiman
Anala V. Shetty, Clifford J. Steer, Walter C. Low
Qin Xie, Haibo Wu, Jiaxin Qiu, Junbo Liu, Qifeng Lyu, Hui Long, Wenzhi Li, Shuo Zhang, Xueyi Jiang, Yuxiao Zhou, Yining Gao, Aaron J. W. Hsueh, Yanping Kuang, Lun Suo
Jeffrey C. Medley, Roberto Perales, Belén Gaete Humada, Katja Doerfel, Ariana Levine, Ganesh Panzade, Christopher M. Hammell, Anna Zinovyeva
A parent-of-origin effect on embryonic telomere elongation determines telomere length inheritance
Hyuk-Joon Jeon, Mia T. Levine, Michael A. Lampson
Alexander Stanton, Selcan Aydin, Daniel A. Skelly, Dylan Stavish, Kim Leonhard, Seth Taapken, Erik McIntire, Matthew Pankratz, Anne Czechanski, Tenneille Ludwig, Ted Choi, Steven P. Gygi, Ivana Barbaric, Steven C. Munger, Laura G. Reinholdt, Martin F. Pera
Natalie E. Toothacre, Kiara L. Rodríguez-Acevedo, Keenan J. Wiggins, Christopher D. Scharer, Montserrat C. Anguera
Rajini Chandrasegaram, Antony M. Hynes-Allen, Beitong Gao, Abhilesh Dhawanjewar, Michele Frison, Stavroula Petridi, Patrick F. Chinnery, Hansong Ma, Jelle van den Ameele
Selcan Aydin, Daniel A. Skelly, Hannah Dewey, J. Matthew Mahoney, Ted Choi, Laura G. Reinholdt, Christopher L. Baker, Steven C. Munger
Satoshi Mashiko, Takuto Yamamoto, Naojiro Minami, Shuntaro Ikeda, Shinnosuke Honda
| Stem cells, regeneration & disease modelling
Xiaohang Long, Dandan CAO, See-Wing Chan, Suyu Hao, Lingxiao Liu, Wai-Yee Chan, Hoi-Hung Cheung
Mizuho Ishikawa, Yen Xuan Ngo, Ikuto Nishikawa, Hiroko Kato, Ryo Maeda, Ryosuke Mizuno, Jun Mizuno, Kenji Izumi, Hiromi Yanagisawa, Aiko Sada
The epigenetic factor Zrf1 regulates intestinal stem cell proliferation during midgut regeneration
Joshua Shin Shun Li, Ying Liu, Ah-Ram Kim, Mujeeb Qadiri, Jun Xu, Baolong Xia, Richard Binari, John M Asara, Yanhui Hu, Norbert Perrimon
Tanusri Roy, Swetlana Ghosh, Niyati Piplani, Lakshmi Kavitha Sthanam, Niharika Tiwary, Sayak Dhar, W. Chingmei Wangsa Konyak, Santosh Surendra Panigrahi, Priya Singh, Divya Tej Sowpati, Sreelaja Nair, Sushil Kumar, P. Chandra Shekar, Shamik Sen
The AMPKα2/PHF2 axis is critical for turning over lipid droplets during muscle stem cell fate
Delia Cicciarello, Sandrine Mouradian, Mélodie Pitchecanin, William Jarassier, Anita Kneppers, Rémi Mounier, Laurent Schaeffer, Isabella Scionti
Gonzalo N. Morales Chaya, Mubarak Hussain Syed
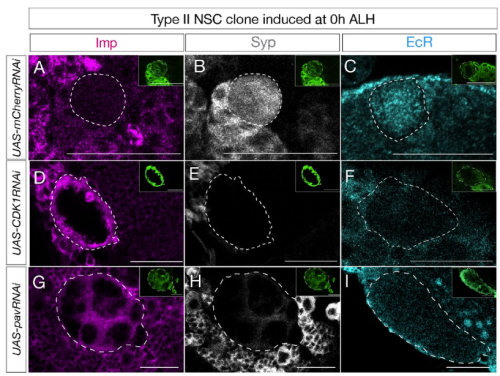
Silvia Da Pra, Stefano Boriati, Carmen Miano, Irene Del Bono, Francesca Sacchi, Chiara Bongiovanni, Alla Aharonov, Nicola Pianca, Riccardo Tassinari, Carlo Ventura, Mattia Lauriola, Eldad Tzahor, Gabriele D’Uva
The central clock drives metabolic rhythms in muscle stem cells
Valentina Sica, Jacob G Smith, Oleg Deryagin, Eva Andres, Vera Lukesova, Mirijam Egg, Nina Cabezas-Wallscheid, Salvador Aznar Benitah, Antonio L. Serrano, Eusebio Perdiguero, Pura Muñoz-Cánoves
Yuanyuan Qin, Parth Chhetri, Elizabeth Theusch, Grace Lim, Sheila Teker, Yu-Lin Kuang, Shahrbanoo Keshavarz Aziziraftar, Mohammad Hossein Mehraban, Antonio Munoz-Howell, Varun Saxena, Dounia Le Guillou, Aras N. Mattis, Jacquelyn J. Maher, Marisa W. Medina
Lauren F. Kunselman, Elaine C. Seaver
ECM Signatures Reveal Quiescent Stem Cell Diversity in the Colonic Niche
Séamus E. Hickey, Massimo Andreatta, Christina Enright, Emmanuel Boucrot, Patrick Kiely, Siobhán B. Cashman, Saintiago J. Carmona, Kieran McGourty
Sarah Stucchi, Lessly P. Sepulveda-Rincon, Camille Dion, Gaja Matassa, Alessia Valenti, Cristina Cheroni, Alessandro Vitriolo, Filippo Prazzoli, George Young, Marco Tullio Rigoli, Martina Ciprietti, Benedetta Muda, Zoe Heckhausen, Petra Hajkova, Nicolò Caporale, Giuseppe Testa, Harry G. Leitch
Ilke Sari, Beliz Uzun, Melda O. Oguz, Eren Gursoy, Nurmuhammet Satlykov, Dila A. Gemalmayan, Nagihan Gonullu, Tomas Valenta, Fatima Aerts Kaya, Merve Gizer, Petek Korkusuz, Bahar Degirmenci
Sara Rigney, Joshua R. York, Carole LaBonne
Secreted Frizzled-Related Protein 1a regulates hematopoietic development in a dose-dependent manner
Amber D. Ide, Kelsey A. Carpenter, Mohamed Elaswad, Katherine Opria, Kendersley Marcellin, Carla Gilliland, Stephanie Grainger
Visakuo Tsurho, Carla Gilliland, Jessica Ensing, Elizabeth Vansickle, Nathan J. Lanning, Paul R. Mark, Stephanie Grainger
Mariana A. Branco, Mafalda Marques Nunes, Ana Luísa Rayagra, Miguel F. Tenreiro, Joaquim M.S. Cabral, Maria Margarida Diogo
Distinct origin and fate for fetal hematopoietic progenitors
F. Soares-da-Silva, G. Nogueira, Marie-Pierre Mailhe, L. Freyer, A. Perkins, S. Hatano, Y. Yoshikai, P. Pereira, A. Bandeira, R. Elsaid, E. Gomez-Perdiguero, A. Cumano
Sema Elif Eski, Jiarui Mi, Macarena Pozo-Morales, Gabriel Garnik Hovhannisyan, Camille Perazzolo, Rita Manco, Imane Ez-Zammoury, Dev Barbhaya, Anne Lefort, Frédérick Libert, Federico Marini, Esteban N. Gurzov, Olov Andersson, Sumeet Pal Singh
Chiari II brain malformation is secondary to open spina bifida
Maryam Clark, Timothy J. Edwards, Dawn Savery, Gabriel L. Galea, Nagaraj Samy, Erwin Pauws, Nicoletta Kessaris, Nicholas D.E. Greene, Andrew J. Copp
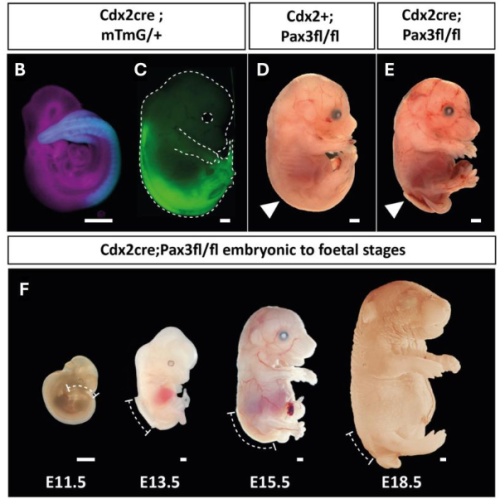
Intestinal stem cell renewal controlled by capillary morphogenesis gene 2 following injury
Lucie Bracq, Audrey Chuat, Béatrice Kunz, Olivier Burri, Romain Guiet, F. Gisou van der Goot
Tae Wan Kim, Jinghua Piao, Vittoria D Bocchi, So Yeon Koo, Se Joon Choi, Fayzan Chaudhry, Donghe Yang, Hyein S Cho, Emiliano Hergenreder, Lucia Ruiz Perera, Subhashini Joshi, Zaki Abou Mrad, Nidia Claros, Shkurte Ademi Donohue, Anika K. Frank, Ryan Walsh, Eugene V. Mosharov, Doron Betel, Viviane Tabar, Lorenz Studer
Camille Curantz, Gabriel Krasovec, Helen R Horkan, Laura Ryan, Áine Varley, Uri Frank
Sharanya Premraj, Poonam Sharma, Mansi Chaudhary, Pooja Shukla, Kshitiz Yadav, Omkar Mahadeo Desai, Rajesh Ramachandran
Andrzej Kubiak, Natalia Bryniarska-Kubiak, Mehmet Eren, Kacper Kowalski, Kinga Gawlińska, Patrycja Kwiecińska, Martine Biarnes-Pelicot, Marie Dessard, Jana El Husseiny, Ti-Thien N-Guyen, Pawel Kożuch, Olga Lis, Marta Targosz-Korecka, Pierre-Henri Puech, Krzysztof Szade
Meiricris Tomaz da Silva, Aniket S. Joshi, Ashok Kumar
Antonella F.M. Dost, Katarína Balážová, Carla Pou Casellas, Lisanne M. van Rooijen, Wisse Epskamp, Gijs J.F. van Son, Willine J. Wetering, Carmen Lopez-Iglesias, Harry Begthel, Peter J. Peters, Niels Smakman, Johan H. van Es, Hans Clevers
Nucleoredoxin regulates WNT signaling during pituitary stem cell differentiation
Michelle Brinkmeier, Leonard Y.M. Cheung, Sean P. O’Connell, Diana K Gutierrez, Eve C. Rhoads, Sally A. Camper, Shannon William Davis
BubR1 and Mad2 regulate adult midgut remodeling in Drosophila diapause
Yuya Adachi, Hiroki Nagai, Takako Fujichika, Aya Takahashi, Masayuki Miura, Yu-ichiro Nakajima
Estrogen-Related Receptor is Required in Adult Drosophila Females for Germline Stem Cell Maintenance
Anna B. Zike, Madison G. Abel, Sophie A. Fleck, Emily D. DeWitt, Lesley N. Weaver
Miao Zhen, Juntao Xie, Rui Yang, Lijuan Liu, Hengdeng Liu, Xuefeng He, Suyue Gao, Junyou Zhu, Jingting Li, Bin Shu, Peng Wang
A niche-dependent redox rheostat regulates epithelial stem cell fate in the distal colon
Xi Chen, Krishnan Raghunathan, Bin Bao, Elsy Ngwa, Andrew Kwong, Zhongyang Wu, Stephen Babcock, Clara Baek, George Ye, Anoohya Muppirala, Qianni Peng, Michael Rutlin, Mantu Bhaumik, Daping Yang, Daniel Kotlarz, Unmesh Jadhav, Meenakshi Rao, Eranthie Weerapana, Xu Zhou, Jose Ordovas-Montanes, Scott B. Snapper, Jay R. Thiagarajah
Ying Ru, Meng Ma, Xianxiao Zhou, Divya Kriti, Ninette Cohen, Sunita D’Souza, Christoph Schaniel, Susan M. Motch Perrine, Sharon Kuo, Oksana Pichurin, Dalila Pinto, Genevieve Housman, Greg Holmes, Eric Schadt, Harm van Bakel, Bin Zhang, Ethylin Wang Jabs, Meng Wu
Glycan-Mediated Mechanosensing Regulates Megakaryocyte-Biased Hematopoietic Stem Cell Subsets
Alejandro Roisman, Leonardo Rivadeneyra, Lindsey Conroy, Melissa M. Lee-Sundlov, Natalia Weich, Simon Glabere, Shikan Zheng, Katelyn E. Rosenbalm, Mark Zogg, George Steinhardt, Anthony J. Veltri, Joseph T. Lau, Tongjun Gu, Hartmut Weiler, Ramon C. Sun, Karin M. Hoffmeister
J.D. Frenster, S. Babin, J.B. Josende Garcia, P. Casani Galdon, P. Pascual-Mas, G. Robertson, J. Garcia Ojalvo, A. Martinez Arias
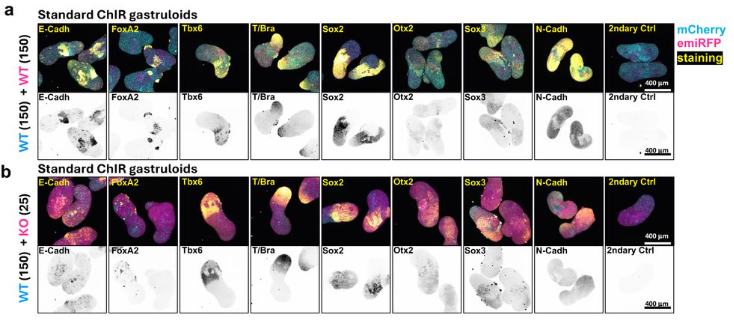
Pancreatic injury induces β−cell regeneration in axolotl
Connor J. Powell, Hani D. Singer, Ashley R. Juarez, Ryan T. Kim, Duygu Payzin-Dogru, Aaron M. Savage, Noah J. Lopez, Steven J. Blair, Adnan Abouelela, Anita Dittrich, Stuart G. Akeson, Miten Jain, Jessica L. Whited
Ashley Rich, Ziqi Lu, Alessandro De Simone, Lucas Garcia, Jacqueline Janssen, Kazunori Ando, Jianhong Ou, Massimo Vergassola, Kenneth D. Poss, Stefano Di Talia
Selection of pre-leukemic hematopoietic stem cells driven by distinct extracellular matrix molecules
Maria Jassinskaja, Daniel Bode, Monika Gonka, Theodoros I Roumeliotis, Alexander J Hogg, Juan A Rubio Lara, Ellie Bennett, Joanna Milek, Bart Theeuwes, M S Vijayabaskar, Lilia Cabrera Cosme, James L C Che, Sandy MacDonald, Sophia Ahmed, Benjamin A Hall, Grace Vasey, Helena Kooi, Miriam Belmonte, Mairi S Shepherd, William J Brackenbury, Iwo Kucinski, Satoshi Yamazaki, Andrew N Holding, Alyssa H Cull, Nicola K Wilson, Berthold Göttgens, Jyoti Choudhary, David G Kent
Regulation of hematopoietic stem cell (HSC) proliferation by Epithelial Growth Factor Like-7 (EGFL7)
Rohan Kulkarni, Chinmayee Goda, Alexander Rudich, Malith Karunasiri, Amog P Urs, Yaphet Bustos, Ozlen Balcioglu, Wenjun Li, Sadie Chidester, Kyleigh A Rodgers, Elizabeth AR Garfinkle, Ami Patel, Katherine E Miller, Phillip G Popovich, Shannon Elf, Ramiro Garzon, Adrienne M Dorrance
| Plant development
Yiru Xu, Heng Zhou, Xiaojiang Wu, Wuyu Cui, Shouling Xu, Xi He, Dan Xiang, Ming Zhou, Lilan Hong
Freya Maria Rosemarie Ziegler, Amelia Gaston, Karine Guy, Marie Devers, Erika Krueger, Bastienne Brauksiepe, Klaus Eimert, Sonia Osorio, Beatrice Denoyes, Bjoern Usadel
Decoding cellular transcriptional regulatory networks governing wheat inflorescence development
Xuemei Liu, Xuelei Lin, Jingmin Kang, Katie A. Long, Jingjing Yue, Chuan Chen, Dongzhi Wang, Ashleigh Lister, lain C. Macaulay, Xin Liu, Cristobal Uauy, Jun Xiao
Li’ang Yu, Giovanni Melandri, Anna C. Dittrich, Sebastian Calleja, Kyle Palos, Aparna Srinivasan, Emily K Brewer, Riley Henderson, Ciara Denise Garcia, Xiaodan Zhang, Bruno Rozzi, Andrea Eveland, Susan J. Schroeder, David Stern, Aleksandra Skirycz, Eric Lyons, Elizabeth A. Arnold, Brian D. Gregory, Andrew D. L. Nelson, Duke Pauli
Robin Schulz, Günter Theißen
Glenn Philippe, Iben Sørensen, Aurore Guérault, Marcella J. Cross, David S. Domozych, Mads H. Clausen, Jocelyn K. C. Rose
Chloroplast genome editing of Rubisco boosts photosynthesis and plant growth
Wataru Yamori, Issei Nakazato, Qu Yuchen, Yukina Sanga, Tomoko Miyata, Ryo Uehara, Yuma Noto, Keiichi Namba, Hiroshi Fukayama, Hiroyoshi Matsumura, Shin-ichi Arimura
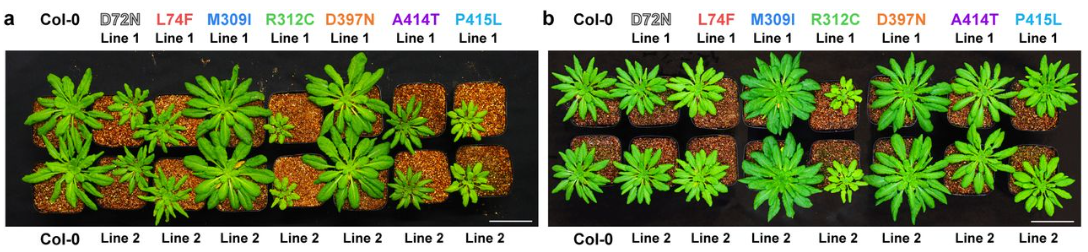
Differences in apple fruit shape are independent of fruit size
Kylie DeViller, Daniel H. Chitwood, Sean Myles, Mao Li, Zoë Migicovsky
Genome-scale transcriptome augmentation during Arabidopsis thaliana photomorphogenesis
Geoffrey Schivre, Lea Wolff, Filippo Maria Mirasole, Elodie Armanet, Mhairi L..H Davidson, Adrien Vidal, Delphine Cumenal, Marie Dumont, Mickael Bourge, Celia Baroux, Clara Bourbousse, Fredy Barneche
Benoit Landrein, Katie Abley, Pau Formosa-Jordan, Elliot M Meyerowitz, Henrik Jonsson, James C.W. Locke
Suzuka Kikuchi, Takumi Kotaka, Yuga Hanaki, Minako Ueda, Takumi Higaki
An unexplored diversity for adaptation of germination to high temperatures in Brassica species
M. Tiret, M.-H. Wagner, L. Gay, E. Chenel, A. Dupont, C. Falentin, F. Gavory, K. Labadie, S. Ducournau, A.-M. Chèvre
| Environment, evolution and development
Albert Chen, Elizabeth M. Steell, Roger B. J. Benson, Daniel J. Field
Defining the cell and molecular origins of the primate ovarian reserve
Sissy E. Wamaitha, Ernesto J. Rojas, Francesco Monticolo, Fei-man Hsu, Enrique Sosa, Amanda M. Mackie, Kiana Oyama, Maggie Custer, Melinda Murphy, Diana J. Laird, Jian Shu, Jon D. Hennebold, Amander T. Clark
Postnatal interaction of size and shape in the human endocranium and brain structures
Kuranosuke Takagi, Osamu Kondo
Evidence for evolution of a new sex chromosome within the Marchantiales haploid plant lineage
Yuan Fu, Xiaoxia Zhang, Tian Zhang, Wenjing Sun, Wenjun Yang, Yajing Shi, Jian Zhang, Qiang He, Deborah Charlesworth, Yuannian Jiao, Zhiduan Chen, Bo Xu
Natasha S. Vitek, Ella Saks, Amy Dong, Robert W. Burroughs, Devin L Ward, Emma Pomeroy, Malgorzata Martin-Gronert, Susan E. Ozanne
Somatic evolution of stem cell mutations in long-lived plants
Frank Johannes
Ayse Tenger-Trolander, Ezra Amiri, Valentino Gantz, Chun Wai Kwan, Sheri A Sanders, Urs Schmidt-Ott
Macroevolution of fly wings proceeds along developmental lines of least resistance
Patrick T. Rohner, David Berger
Rebecca von Hellfeld, Rebecca Konietzny, Philip D. Charles, Roman Fischer, Benedikt M. Kessler, Stuart Wigby, Irem Sepil, Juliano Morimoto
Mei Liang, Lee Ringham, Changning Ye, Xu Yan, Nathan Schaumburger, Mikolaj Cieslak, Michael Blinov, Przemyslaw Prusinkiewicz, Yao-Wu Yuan
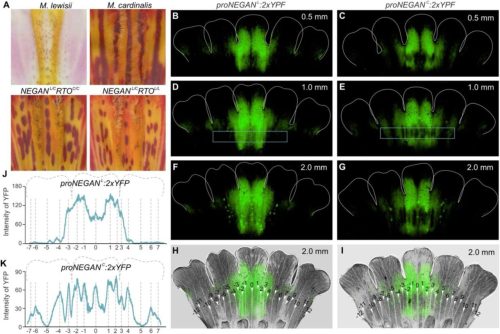
Thomas S. O’Leary, Emily E. Mikucki, Sumaetee Tangwancharoen, Joseph R. Boyd, Seth Frietze, Sara Helms Cahan, Brent L. Lockwood
Hieu Vo, C. Ben Lovely
Chaetognaths exhibit the most extensive repertoire of Hox genes among protostomes.
June F. Ordonez, Tim Wollesen
Gene regulatory mechanisms underlying evolutionary adaptations of homologous neuronal cell types
Nuria Flames, Andrea Millan-Trejo, Carlos Mora-Martinez, Adrian Tarazona-Sanchez, Carla Lloret-Fernandez, Rafael Alis, Antonio Jordan, Arantza Barrios
Isabella Joyce, Austen A. Barnett
Divergent morphologies with convergent performance in the mandible of pelagiarian fishes
Andrew Knapp, Gizeh Rangel-de Lazaro, Matt Friedman, Zerina Johanson, Kory M Evans, Sam Giles, Hermione T Beckett, Anjali Goswami
Dataset on Temperature Dependency of Zebrafish Early Development
Angelina Miller, Katja Lisa Schröder, Karsten Eike Braun, Caitlin Steindorf, Richard Ottermanns, Martina Roß-Nickoll, Thomas Backhaus
Molecular basis of arthropod appendage diversity
Heather S. Bruce, Nipam H. Patel
Kip D. Lacy, Jina Lee, Kathryn Rozen-Gagnon, Wei Wang, Thomas S. Carroll, Daniel J.C. Kronauer
Mark E. Corkins, Adrian Romero-Mora, MaryAnne A. Achieng, Nils O. Lindström, Rachel K. Miller
John W. Terbot II, Vivak Soni, Cyril J. Versoza, Mark Milhaven, Adriana Calahorra-Oliart, Devangana Shah, Susanne P. Pfeifer, Jeffrey D. Jensen
John W. Terbot II, Vivak Soni, Cyril J. Versoza, Mark Milhaven, Adriana Calahorra-Oliart, Devangana Shah, Susanne P. Pfeifer, Jeffrey D. Jensen
Arsenii I. Kairov, Vitaly V. Kozin
Inverse hourglass pattern of conservation in rodent molar development
Jérémy Ganofsky, Mathilde Estevez-Villar, Marion Mouginot, Sébastien Moretti, Marion Nyamari, Marc Robinson-Rechavi, Sophie Pantalacci, Marie Sémon
Cell Biology
Follicular mural granulosa cells stockpile glycogen to fuel corpus luteum pre-vascularization
Jianning Liao, Qinghua Liu, Cong Liu, Guiqiong Liu, Xiang Li, Xiaodong Wang, Yaqin Wang, Ruiyan Liu, Hao Wu, Hongru Shi, Yongheng Zhao, Wenkai Ke, Zaohong Ran, Zian Wu, Bowen Tan, Chaoli Wang, Xunping Jiang, Quanfeng Wang, Qingzhen Xie, Guoshi Liu, Changjiu He
Neha Tiwari, Madhu Gwaldas Tapadia
Vanesa Fernández-Majada, Jordi Comelles, Aina Abad-Lázaro, Verónica Acevedo, Anna Esteve-Codina, Xavier Hernando-Momblona, Eduard Batlle, Elena Martinez
Protein phosphatase 4 is required for centrosome asymmetry in fly neural stem cells
Roberto Carlos Segura, Emmanuel Gallaud, Adam von Barnau Sythoff, Kumar Aavula, Jennifer A. Taylor, Danielle Vahdat, Jan Pielage, Clemens Cabernard
Rebekah Niewoehner, David Paulding, Jesus Leal, Rolf W. Stottmann
Functional characterization of eicosanoid signaling in Drosophila development
Daiki Fujinaga, Cebrina Nolan, Naoki Yamanaka
Wnt/LRP6 signaling imbalance impairs ciliogenesis in human retina epithelial cells
Cheng Yuan, Ayushi Branushali, Matias Simons, Sergio P. Acebrón, Gislene Pereira
CONSERVED NUCLEAR MORPHOLOGY IDENTIFIES FUNCTIONAL RADIAL GLIA NEURAL PROGENITORS
J.P. Soriano, C. Borau, R. Sortino, L. Garma, J.A. Ortega, J. Asín, S. Alcántara
Yasaman Heidarian, Tess D. Fasteen, Liam Mungcal, Kasun Buddika, Nader H. Mahmoudzadeh, Travis Nemkov, Angelo D’Alessandro, Jason M. Tennessen
Yi Li, Ida Calvi, Monica Gotta
Chemokine induces phase transition from non-directional to directional migration during angiogenesis
Ning Gui, Keisuke Sako, Moe Fukumoto, Naoki Mochizuki, Hiroyuki Nakajima
William M. Salvidge, Chris Brimson, Nicole Gruenheit, Li-Yao Huang, Catherine J. Pears, Jason B. Wolf, Christopher R.L. Thompson
Benjamin Wetherall, David Bulmer, Alexandra Sarginson, Christopher Thomas, Suzanne Madgwick
Linan Gao, Roman Franěk, Tomáš Tichopád, Marek Rodina, David Gela, Radek Šindelka, Taiju Saito, Martin Pšenička
Manisha Goyal, Sakshi Tiwari, Bruce Cooper, Ramaswamy Subramanian, Tina Mukherjee
Intense oocyte competition builds the female reproductive reserve in mice
Hua Zhang, Yan Zhang, Yingnan Bo, Kaixin Cheng, Lu Mu, Jing Liang, Lingyu Li, Xindi Hu, Ge Wang, Kaiying Geng, Xuebing Yang, Wenji Wang, Longzhong Jia, Xueqiang Xu, Jingmei Hu, Chao Wang, Yuwen Ke, Guoliang Xia
Decoding human placental cellular and molecular responses to maternal obesity and fetal growth
Hong Jiang, Emilie Derisoud, Denise Parreira, Nayere Taebnia, Paulo R. Jannig, Reza Zandi Shafagh, Allan Zhao, Congru Li, Macarena Ortiz, Manuel Alejandro Maliqueo, Elisabet Stener-Victorin, Volker M. Lauschke, Qiaolin Deng
Kennosuke Ichikawa, Yuzuha Motoe, Tenkai Watanabe, Ryo Ezaki, Mei Matsuzaki, Hiroyuki Horiuchi, Michael J. McGrew
Centriole Loss in Embryonic Development Disrupts Axonal Pathfinding and Muscle Integrity
Beatriz González, Júlia Sellés-Altés, Jèrica Pla-Parron, Judith Castro-Ribera, Sofia J. Araújo
A sperm–oocyte protein partnership required for egg activation in Caenorhabditis elegans
Tatsuya Tsukamoto, Ji Kent Kwah, Mark E. Zweifel, Naomi Courtemanche, Micah D. Gearhart, Katherine M. Walstrom, Aimee Jaramillo-Lambert, David Greenstein
Sagar D. Joshi, Timothy R. Jackson, Lin Zhang, Carsen Stuckenholz, Lance A. Davidson
Modelling
The evolution of mutation rates in the light of development and cell-lineage selection
Paco Majic, Malvika Srivastava, Justin Crocker
Strategies for resolving cellular phylogenies from sequential lineage tracing data
Nicola Mulberry, Tanja Stadler
Self-renewal without niche instruction, feedback or fine-tuning
Ben D MacArthur, Philip Greulich
Morphogen Patterning in Dynamic Tissues
Alex M. Plum, Mattia Serra
Mathematical modelling of tissue growth control by a negative feedback
B. Kazmierczak, V. Volpert
STOCHASTIC MODELING OF HEMATOPOIETIC STEM CELL DYNAMICS
Carlos Alfaro-Quinde, Katerina E. Krstanovic, Paula A. Vásquez, Katie L. Kathrein
The Network Basis of Pattern Formation: A Topological Atlas of Multifunctional Turing Networks
Laura Regueira López de Garayo, Luciano Marcon
Tools & Resources
Zifan Gu, Rashi Gupta, David E Cantonwine, Thomas F McElrath, Henning Tiemeier, James Selib Michaelson
A method for inducing a senescence trend in mesenchymal stem cells using D-galactose
Xiaoming Ji, Xiaoyu Zhou, Tingitng Chen, Chunchun Duan, Wenjing Tian, Liyang Zhu, Yan Ma, Liyang Gao
Paula R Brown, Martin A Estermann, Artiom Gruzdev, Gregory J Scott, Thomas B Hagler, Manas K Ray, Humphrey Hung-Chang Yao
Giada Rossignoli, Michael Oberhuemer, Ida Sophie Brun, Irene Zorzan, Anna Osnato, Anne Wenzel, Emiel van Genderen, Andrea Drusin, Giorgia Panebianco, Nicolò Magri, Mairim Alexandra Solis, Chiara Colantuono, Sam Samuël Franciscus Allegonda van Knippenberg, Thi Xuan Ai Pham, Sherif Khodeer, Paolo Grumati, Davide Cacchiarelli, Paolo Martini, Nicolas Rivron, Vincent Pasque, Jan Jakub Żylicz, Martin Leeb, Graziano Martello
Nagham Khouri-Farah, Emma Wentworth Winchester, Brian M. Schilder, Kelsey Robinson, Sarah W. Curtis, Nathan G. Skene, Elizabeth J. Leslie-Clarkson, Justin Cotney
Zonal endothelial cell heterogeneity underlies murine renal vascular development
Peter M Luo, Neha Ahuja, Christopher Chaney, Danielle Pi, Aleksandra Cwiek, Zaneta Markowska, Chitkale Hiremath, Denise Marciano, Karen K Hirschi, M Luisa Iruela-Arispe, Thomas J Carroll, Ondine Cleaver
Jiarui Mi, Lipeng Ren, Ka-Cheuk Liu, Lorenzo Buttò, Daniel Colquhoun, Olov Andersson
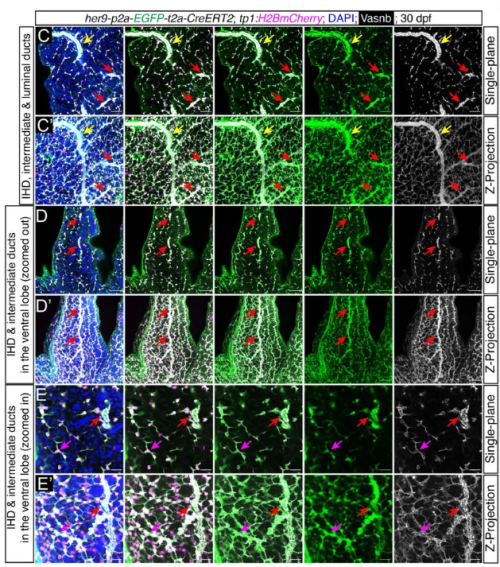
Emily K. Ho, Rebecca P. Kim-Yip, Alison G. Simpkins, Payam E. Farahani, Harrison R. Oatman, Eszter Posfai, Stanislav Y. Shvartsman, Jared E. Toettcher
Isabel Turpin, Adriana Modrego, Andrea Martí-Sarrias, Anna-Christina Haeb, Laura García-González, Jordi Soriano, Núria Ruiz, Irene Peñuelas-Haro, Elisa Espinet, Daniel Tornero, Oscar Lao, Sandra Acosta
Giada Rossignoli, Michael Oberhuemer, Ida Sophie Brun, Irene Zorzan, Anna Osnato, Anne Wenzel, Emiel van Genderen, Andrea Drusin, Giorgia Panebianco, Nicolò Magri, Mairim Alexandra Solis, Chiara Colantuono, Sam Samuël Franciscus Allegonda van Knippenberg, Thi Xuan Ai Pham, Sherif Khodeer, Paolo Grumati, Davide Cacchiarelli, Paolo Martini, Nicolas Rivron, Vincent Pasque, Jan Jakub Żylicz, Martin Leeb, Graziano Martello
Yi Chai, Ruiying Zhang, Shunze Jia, Danfei Zhu, Siyi Chen, Xudong Fu, Xin Sheng
Zhenyi Zhang, Jie Qiao, Yidong Chen, Peijie Zhou